Introduction
The Antarctic Ice Sheet is the largest component (by volume) of Earth’s cryosphere. It has a major impact on both regional and global climate through the modification of surface albedo and by altering atmosphere and ocean circulation (DeConto et al., 2007; Bintanja et al., 2013; Colleoni et al., 2018; Golledge et al., 2019). The Antarctic Ice Sheet is the largest store of freshwater on Earth and has the potential to raise global sea level by 58 m if completely melted. Today, it receives 2,100 Gt of annual snowfall that is balanced by mass lost from ice flowing under gravity toward the coast, where it is removed approximately equally by ocean melting of floating ice shelves and the calving of icebergs (Rignot et al., 2019). Unlike the Greenland Ice Sheet, which has an extensive ablation zone, there is minimal melting on the Antarctic Ice Sheet surface at present, although surface meltwater is found in small areas (Trusel et al., 2013; Kingslake et al., 2017; Lenaerts et al., 2017; Banwell et al., 2019).
Much of the ice sheet (~23 m sea level equivalent) is “marine,” meaning that it sits on bedrock currently below sea level (Fretwell et al., 2013) and is often buttressed by floating ice shelves. There are concerns about the role ocean warming plays regarding the future stability of these parts of the ice sheet (Alley et al., 2015). Of particular concern is the stability of the smaller West Antarctic Ice Sheet if its supporting ice shelves should be lost (Mercer, 1978; Fürst et al., 2016; Pattyn, 2018). There is also growing appreciation that marine portions (~19 m sea level equivalent) of the much larger East Antarctic Ice Sheet may be vulnerable to ocean warming (e.g., Rintoul et al., 2018; Wilson et al., 2018). Although today ~40% of the Antarctic Ice Sheet volume sits on bedrock below sea level, the fraction that is marine has increased through time. This increase is a result of tectonics and glacial evolution, which carved Antarctica’s landscapes, moved sediment to the expanding continental margins, and depressed the bedrock (Bart, 2003; Young et al., 2011; Colleoni et al., 2018; Paxman et al., 2018). Recent reconstructions of past Antarctic bedrock topography for a number of intervals show how the marine fraction of Antarctica has changed through time (Figure 1; Paxman et al., 2019).
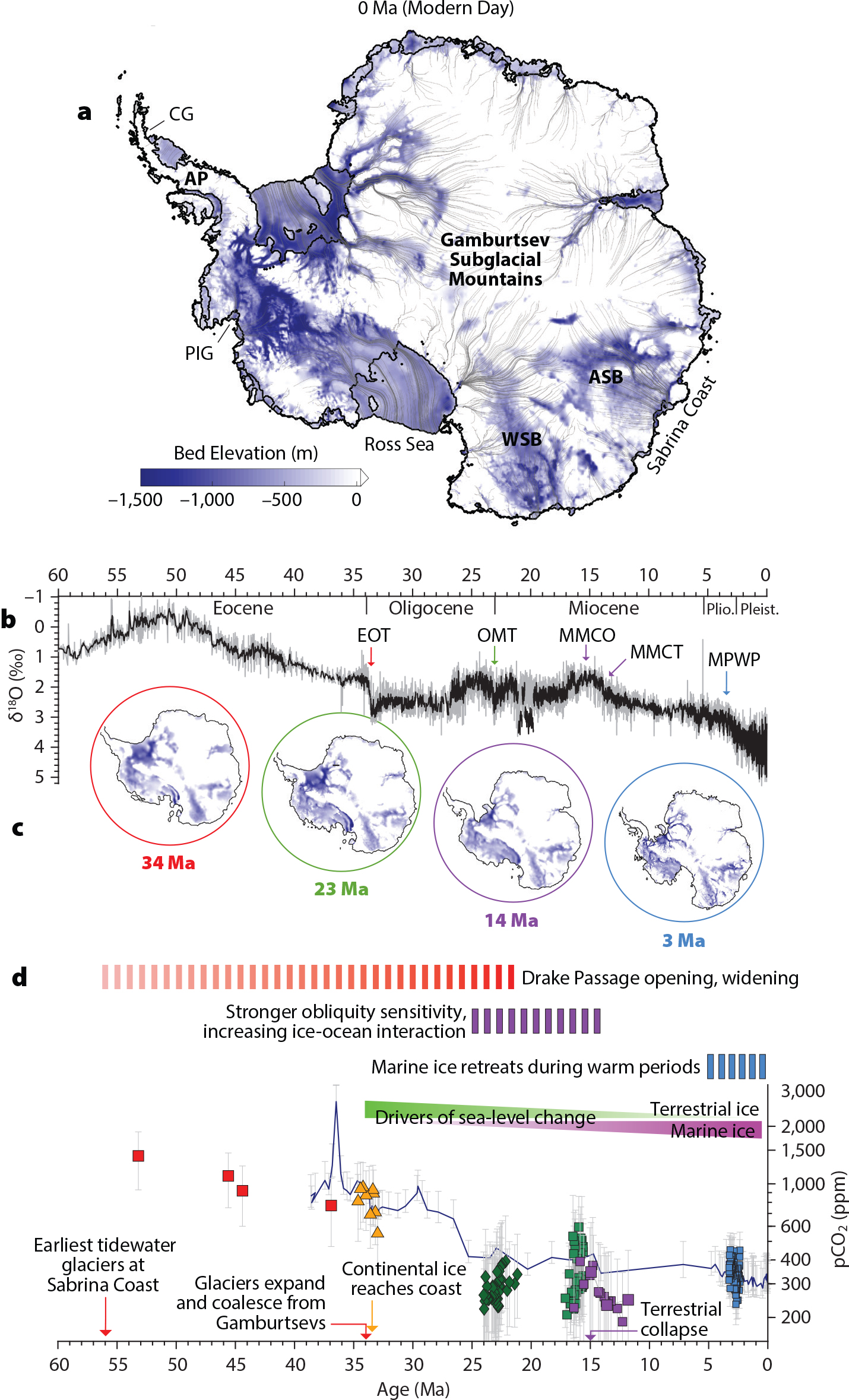
Figure 1. (a) Present-day Antarctica. Blue areas indicate marine ice, with topography currently below sea level, and white areas indicate terrestrial ice, grounded on topography above sea level. The black line is the present-day grounding line, the transition between grounded and floating ice. Gray flowlines indicate direction of surface ice flow from an ice sheet model simulation. AP = Antarctic Peninsula. ASB = Aurora Subglacial Basin. CG = Crane Glacier. PIG = Pine Island Glacier. WSB = Wilkes Subglacial Basin. (b) The composite oxygen isotope record from deep-sea foraminifera reveals the general history of the evolution of the Antarctic Ice Sheet, with higher values for increasing ice volume (Zachos et al., 2008). EOT = Eocene-Oligocene transition. OMT = Oligocene-Miocene transition. MMCO = Mid-Miocene climatic optimum. MMCT = Middle Miocene climate transition. MPWP = Mid-Pliocene warm period. (c) Reconstructions of past marine (blue) and terrestrial (white) ice distribution on Antarctica for different time intervals (Paxman et al., 2019). (d) Composite of selected marine-based proxy CO2 reconstructions (Pearson et al., 2009; Foster et al., 2012; Zhang et al., 2013; Greenop et al., 2014, 2019; Martínez-Botí et al., 2015; Anagnostou et al., 2016). > High res figure
|
The remainder of the Antarctic Ice Sheet is “terrestrial,” that is, grounded on bedrock presently above sea level, so its stability is largely controlled by direct atmospheric melting and buttressing by surrounding ice and the basal topography (e.g., Morlighem et al., 2020). Most importantly, the marine sectors of the ice sheet have the potential to lose mass through ocean-driven melting and iceberg calving, as well as surface melting, whereas the terrestrial sectors lose mass when surface melting exceeds snowfall, or by ice flow. Whether retreat occurs in marine or terrestrial sectors can affect the rate at which mass is lost and therefore the rates at which the ice sheet contributes to sea level change. Understanding the style of past ice sheet retreat is therefore critical to understanding possible rates of future sea level rise (DeConto and Pollard, 2016; Rintoul et al., 2018; Dowdeswell et al., 2020; Golledge, 2020).
Our current understanding of the formation and subsequent waxing and waning of the Antarctic Ice Sheet on million-year timescales is largely based on marine sediment records recovered over the past 50 years through scientific ocean drilling (e.g., Kennett and Shackleton, 1976; Barker et al., 1999; Expedition 318 Scientists, 2010). A recently published review in Oceanography focused on how scientific ocean drilling of marine sedimentary records from the Antarctic continental margin has revolutionized understanding of the past behavior of the Antarctic Ice Sheet (Escutia et al., 2019). The ice proximal records discussed provide critical data in support of far-field records, which track the pacing of ice sheet change through changes in global ice volume and sea level, and also provide information on the climate drivers that caused these changes (e.g., Littler et al., 2019).
Although Antarctica was partially glaciated during intervals of the Eocene, with glaciation in the high Gamburtsev Mountains (Rose et al., 2013) and glaciers reaching the coast during cooler intervals (Gulick et al., 2017), the onset of continental-sized glaciation occurred during the earliest Oligocene (Miller et al, 1991; Zachos et al., 1992). Immediately after the Eocene-Oligocene boundary, ~32.8 million years ago, a continental-scale ice sheet reached the coast of Antarctica (Stocchi et al., 2013; Galeotti et al., 2016). Throughout the Oligocene and into the early Miocene, ice advanced and retreated across the expanding continental shelf, but the magnitude of these oscillations is still subject to debate (McKay et al., 2016; De Vleeschouwer et al., 2017). Although not a direct measure of ice volume, far-field reconstructions based on oxygen isotope records from benthic foraminifera suggest cycles similar in magnitude to that of modern day Antarctic Ice Sheet volume (de Boer et al., 2010; Liebrand et al., 2017; Miller et al., 2020). The pacing of this early Antarctic Ice Sheet gradually shifted to a stronger sensitivity to obliquity (Earth’s axial tilt) toward the mid and then late Miocene, as there was increased ice-ocean interaction (Levy et al., 2019). The ice sheet retreated substantially during the middle Miocene as atmospheric and ocean temperatures increased, and in this warmer and wetter climate, woody plants grew on the Antarctic coast (Warny et al., 2009; Lear et al., 2010; Feakins et al., 2012; Levy et al., 2016; Pierce et al., 2017; Sangiorgi et al., 2018). Immediately following this period, across the middle Miocene climate transition (~14 million years ago), the climate cooled and the ice sheet expanded and became more stable (Shevenell et al., 2004, 2008; Holbourn et al., 2005; Lewis et al., 2008). In the more recent geologic past, the Antarctic Ice Sheet likely retreated during warm intervals of the mid-Pliocene and during some of the warmest interglacials of the late Pleistocene, although this ice was likely sourced only from the marine sectors (Cook et al., 2013; Shakun et al., 2018; Wilson et al., 2018). High sea levels during the last interglacial are often interpreted as evidence for retreat of the Antarctic Ice Sheet, in particular, marine sectors of the West Antarctic Ice Sheet (Dutton et al., 2015; Rohling et al., 2019); however, direct evidence for the loss of the West Antarctic Ice Sheet during the last interglacial is still lacking (e.g., Turney et al., 2020). Note that aspects of this overview are disputed—notably, the magnitude of past Antarctic Ice Sheet retreat has generated many vigorous debates (e.g., Barrett, 2013).
Here, we focus on the separate challenges of simulating retreat of the marine and terrestrial sectors of the ice sheet by drawing on two intervals: (1) the middle Miocene, an interval that occurred ~15 million years ago, when atmospheric CO2 concentrations were similar to those projected for the coming decades under intermediate emissions pathways (Foster et al., 2012), and (2) the mid-Pliocene, an interval that occurred ~3 million years ago and likely the last time that atmospheric CO2 concentrations were as high as they are today (Cook et al., 2013; Martínez-Botí et al., 2015; Shakun et al., 2018). There is evidence for retreat of the terrestrial Antarctic Ice Sheet during the middle Miocene (e.g., Miller et al., 2020), and it is likely that there was retreat of the marine Antarctic Ice Sheet during the mid-Pliocene (Cook et al., 2013; Shakun et al., 2018; Dumitru et al., 2019; Grant et al., 2019). Note that in the rest of this paper, we purposely avoid distinction between the East and West Antarctic Ice Sheets, both of which contain terrestrial and marine sectors.
Terrestrial Ice Sheet Retreat: Complete Collapse of the Antarctic Ice Sheet During the Middle Miocene?
Records from around the Antarctic margin (Levy et al., 2016; Gulick et al., 2017; Pierce et al., 2017) and from far-field sea level and ice volume estimates (Shevenell et al., 2008; Miller et al., 2020) support retreat of the Antarctic Ice Sheet during the warm middle Miocene. The change in ice mass led to sea level change on the order of ~60 m (Kominz et al., 2008; John et al., 2011) and fluctuations in the oxygen isotope composition of seawater, an estimate of ice volume, of ~0.5‰ (Lear et al., 2010). These conditions would have required major retreat of the terrestrial-based ice sheet through surface melting—possibly complete collapse of the ice sheet (Pekar and DeConto, 2006; Miller et al., 2020). However, simulating this retreat with coupled climate and ice sheet models with boundary conditions appropriate for the middle Miocene has been challenging (Pollard and DeConto, 2005). The key middle-Miocene differences in boundary conditions that impact the ice sheets are differences in astronomical parameters, paleogeography (although these differences are relatively small compared with modern), and greenhouse gas concentrations. To generate widespread surface melting and retreat of the terrestrial Antarctic Ice Sheet with global circulation model (GCM)-forced ice sheet models requires a much larger increase in atmospheric CO2 than can be reconstructed from proxy records (Greenop et al., 2014).
The growth of the Antarctic Ice Sheet cooled the Antarctic continent’s climate. Principally, increased albedo reflected more sunlight, and the atmospheric lapse rate led to cooling of the ice surface as the elevation of the growing ice sheet increased (Huybrechts, 1993). These strong positive feedbacks mean that simulating retreat of the Antarctic Ice Sheet requires a magnitude of warming that is inconsistent with proxy reconstructions of atmospheric CO2 during the middle Miocene (Pollard and DeConto, 2005; Langebroek et al., 2009). Although simulations of the onset of Antarctic glaciation are largely consistent with proxy reconstructions (DeConto and Pollard, 2003; Pearson et al., 2009), the simulated deglacial CO2 threshold is much higher than indicated by proxy records and generally outside the error range of these reconstructions (Foster et al., 2012; Foster and Rohling, 2013; shown in red on Figure 2). Following its inception, the simulated Antarctic Ice Sheet is therefore much more stable than the geologic record suggests. This is a fundamental problem because models that cannot capture past collapse will be conservative with respect to projections of future sea level change. Proposed solutions to this conundrum have targeted (a) the proxy records, for example, CO2 was higher than thought in the middle Miocene (Goldner et al., 2014), or we have been misinterpreting ice volume proxies; (b) climate and ice sheet models simulating an overly stable ice sheet (Pollard and DeConto, 2005; Langebroek et al., 2009); (c) a missing forcing, for example, a larger role of changes in ocean gateways and changes in paleogeography; or (d) some combination of these effects (e.g., Langebroek et al., 2009; Gasson et al., 2016b; Stap et al., 2019).
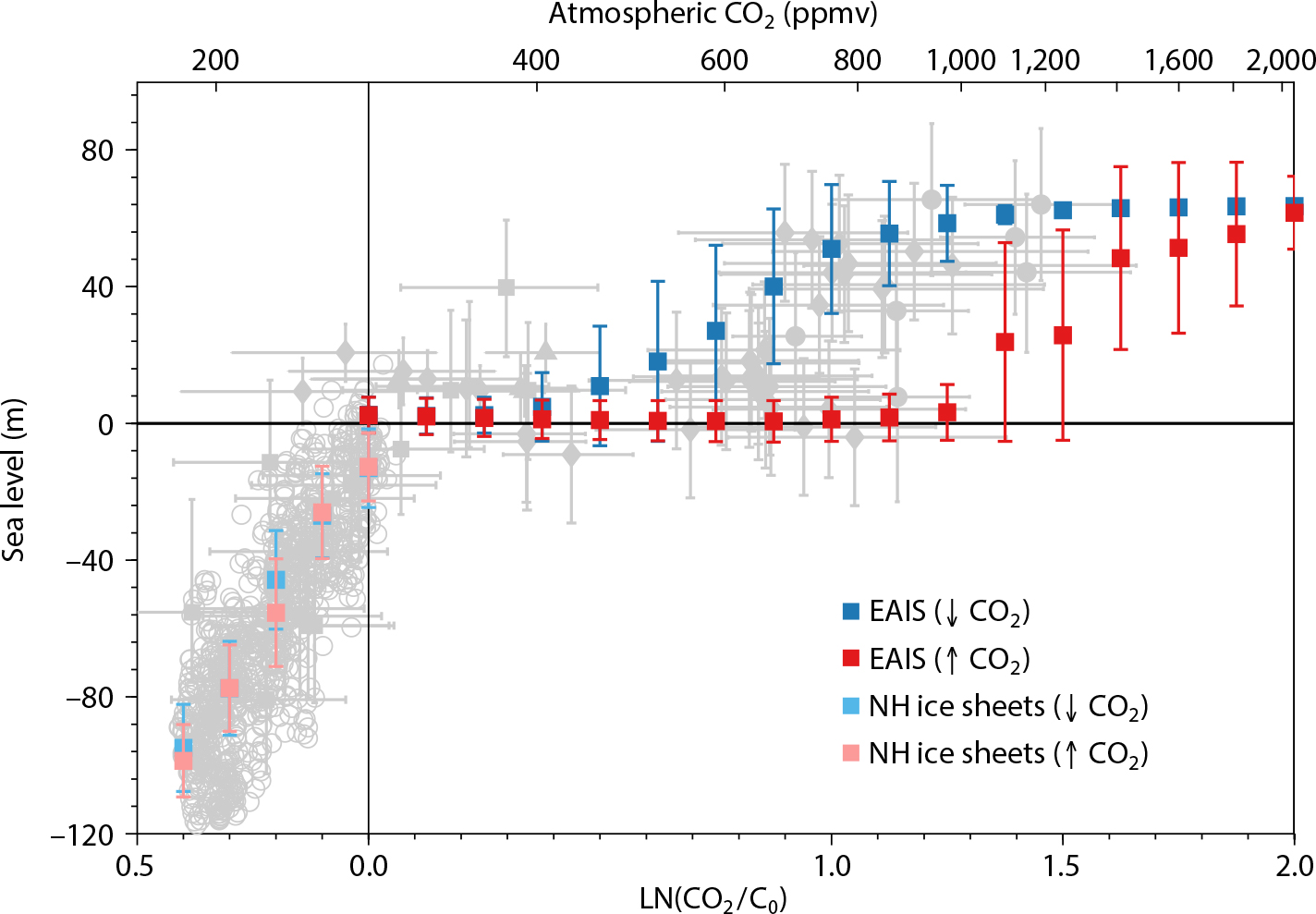
Figure 2. Reconstruction of regional sea level against proxy CO2 for the past 40 million years in gray (Foster and Rohling, 2013, and references therein). Sea level reconstructions are based on a variety of sources and may have local tectonic effects; as such, they should be viewed as relative sea level changes. The red and blue symbols are ice sheet model simulations of the East Antarctic Ice Sheet (EAIS) and Northern Hemisphere (NH) ice sheets; blue indicates a decrease in atmospheric CO2 and red an increase in atmospheric CO2. The error bars represent the binning of a perturbed parameter ensemble of simulations. Note that these ice sheet model simulations lack marine ice sheet physics. > High res figure
|
The large ice volume changes in the Miocene Climate Optimum are based on assumptions inherent to our understanding of the oxygen isotope composition of benthic foraminifera, which provides a record of both global ice volume and deep ocean temperature that must be deconvolved (Shackleton, 1967). Multiple approaches have been used to extract ice volume from the benthic oxygen isotope records, including using an independent temperature proxy (e.g., Shevenell et al., 2008; Lear et al., 2010), estimating ice volume to temperature partitioning (e.g., Liebrand et al., 2017), and inverse modeling (de Boer et al., 2010). Langebroek et al. (2010) noted that producing an accurate ice volume record also requires knowledge of the changes in oxygen isotope composition of the ice sheets through time. Indeed, such changes, which are caused by changes in atmospheric moisture transport and ice sheet height, could lead to an overestimation of past ice volume changes (Winnick and Caves, 2015). Reconstructions that do not take this process into account (e.g., Miller et al., 2020) should be viewed with caution. Similarly, sea level reconstructions from passive continental margins may also contain signals that are caused by mantle dynamic topography rather than eustasy caused by the waxing and waning of the ice sheets (Moucha et al., 2008).
Alternatively, if ice-proximal reconstructions of past ice sheet extent and past estimates of ice volume change are deemed reliable, and sea level did rise and fall by as much as 60 m, then it is important to determine why current climate and ice sheet models are unable to produce this level of dynamism. Improved representation of ice sheet–climate feedbacks is one way of increasing ice sheet dynamism. Recent simulations that used an asynchronous ice sheet–climate coupling and that accounted for changes in ice sheet oxygen isotopes are a better match to benthic oxygen isotope estimates and records from the Antarctic margin (Gasson et al., 2016b; Levy et al., 2016), although they still predict a smaller sea level amplitude (~35 m) than some sea level reconstructions (John et al., 2011; Miller et al., 2020). These simulations also include a mechanism for the structural failure of marine ice cliffs, discussed in more detail below.
The problem of simulating the retreat of terrestrial Antarctic ice is linked to another well-studied model-data disagreement in paleoclimate (Barron, 1983)—the reduced temperature gradient between the poles and the tropics during warm periods and, in particular, the strong polar warming shown by temperature proxies that is generally not matched in coupled ocean-atmosphere model simulations (cf. Huber and Caballero, 2011; Sagoo et al., 2013). Successive generations of GCMs have failed to capture this polar amplification (Lunt et al., 2012); the models that come closest to the proxies require unrealistically high CO2 forcing or model tuning. However, recent results from the latest generation of GCMs show promise for resolving this long-standing problem (Lunt et al., 2020).
The reduced equator-to-pole temperature gradient is perhaps best associated with the early Eocene, a warm interval when there were no ice sheets on Antarctica. Improvements to climate model cloud physics has led to enhanced Eocene warming at high latitudes through shortwave cloud feedbacks; these results are closer to proxy-derived temperature reconstructions at high latitudes (Zhu et al., 2019), although they may now be too warm in the tropics (Zhu et al., 2020). The new generation of climate models includes a subset of models that have much higher climate sensitivity (the amount of warming for a doubling of atmospheric CO2) than earlier models, in part because of developments in the representation of cloud physics (Zelinka et al., 2020). Paleoclimate data are a key test as to whether this higher climate sensitivity is plausible or not (Zhu et al., 2020).
It remains to be seen how the presence of significant ice on Antarctica affects polar amplification and shortwave cloud feedbacks in this new generation of climate models, something missing in studies of the early Eocene. Another modeling target is the Miocene. The advantage of focusing on the Miocene is that it is an interval during which the global continental configuration was fairly similar to today—importantly including the presence of ice on Antarctica (Goldner et al., 2014). It is possible that with greater polar amplification there will be enhanced surface melting and a strong surface mass balance feedback as the ice elevation decreases and melt accelerates at a lower atmospheric CO2 threshold than previously simulated. Idealized simulations have shown that the ice sheet hysteresis problem can be reduced with an increase in polar amplification, although a mechanism to drive this increase is lacking (Langebroek et al., 2009). It is therefore an exciting time to reassess the longstanding problem of simulating past changes to the terrestrial sectors of the Antarctic Ice Sheet.
There is still much work to be done to understand how the Antarctic Ice Sheet responded to past climate changes and, in particular, what drove past retreat of terrestrial ice. Advances will come from a combined approach targeting both far-field and ice-proximal data (Kennicutt et al., 2015). Clearly defined modeling targets, such as quantitative ice-volume estimates or locations with evidence for meltwater, are incredibly useful (e.g., Lewis et al., 2006; Warny et al., 2009; Mudelsee et al., 2014; Gulick et al., 2017). The recent retrieval of new ice-proximal records from three sectors of Antarctica as part of the International Ocean Discovery Program are already providing some of these key records (Escutia et al., 2019; McKay et al., 2019). If the retreat of the terrestrial Antarctic Ice Sheet through surface melting did occur under modest atmospheric CO2 concentrations, we may be forced to reassess long-term future projections (on millennial timescales) of the response of the ice sheet to anthropogenic warming (e.g., Winkelmann et al., 2015).
Marine Ice Sheet Retreat: Ice Sheet Response to Mid-Pliocene Warmth
During the middle Miocene Climate Transition, the climate cooled and the terrestrial Antarctic Ice Sheet became more stable (Kennett, 1977; Shevenell et al., 2004). This scenario is supported by cosmogenic isotope data from the Ross Sea suggesting that for the past 8 million years there has been no retreat of the terrestrial Antarctic Ice Sheet (at least of the sectors draining through the Ross Sea) that would expose land (Shakun et al., 2018). Any ice loss that raised sea levels during this interval would have come from the marine sectors of Antarctica, such as the West Antarctic Ice Sheet, the large basins of East Antarctica, and the Wilkes and Aurora subglacial basins (shown in Figure 1). As mentioned previously, these sectors contain enough ice to raise global sea level by ~23 m. This figure is similar to some estimates of sea level rise during the mid-Pliocene warm interval (3.0–3.2 million years ago; Miller et al., 2012), the last time atmospheric CO2 concentrations exceeded 400 ppm (Martínez-Botí et al., 2015). The sea level maximum during the mid-Pliocene remains poorly constrained, with large uncertainties (Dutton et al., 2015). However, there is physical evidence for substantial ice retreat in the marine sectors of East Antarctica (Cook et al., 2013) as well as far-field evidence for >10 m sea level fluctuations (Dumitru et al., 2019; Grant et al., 2019).
Marine ice sheets have long been of interest because of their potential vulnerability to ocean warming (Mercer, 1978). A grounded ice sheet and a floating ice shelf connect at the grounding line. The rate of ice flow from grounded to floating ice is very sensitive to the ice thickness at the grounding line. Analytical solutions suggest that the ice flow across the grounding line increases highly nonlinearly in response to increases in the grounding line ice thickness. In places where the ice sheet bed is on a slope that deepens upstream of the grounding line, including in the large marine basins mentioned above, the geometry of the ice sheet creates the potential for a runaway retreat (Schoof, 2007). As the grounding line retreats backward across this deepening bed, a positive feedback called the “Marine Ice Sheet Instability” (MISI) develops because of the strong increase in ice flow (Mercer, 1978). Restabilization only occurs when the profile of the bed topography changes and the grounding line retreats to sufficiently shallow topography (Figure 3a; Alley and Joughin, 2012). The ability for ice sheet models to correctly simulate the MISI is a key test conducted in inter-model comparison projects (Cornford et al., 2020). The mid-Pliocene warm interval could be considered a real-world test of marine ice sheet behavior, with the caveats that the forcing and the response both have uncertainties (DeConto and Pollard, 2016; Dolan et al., 2018).
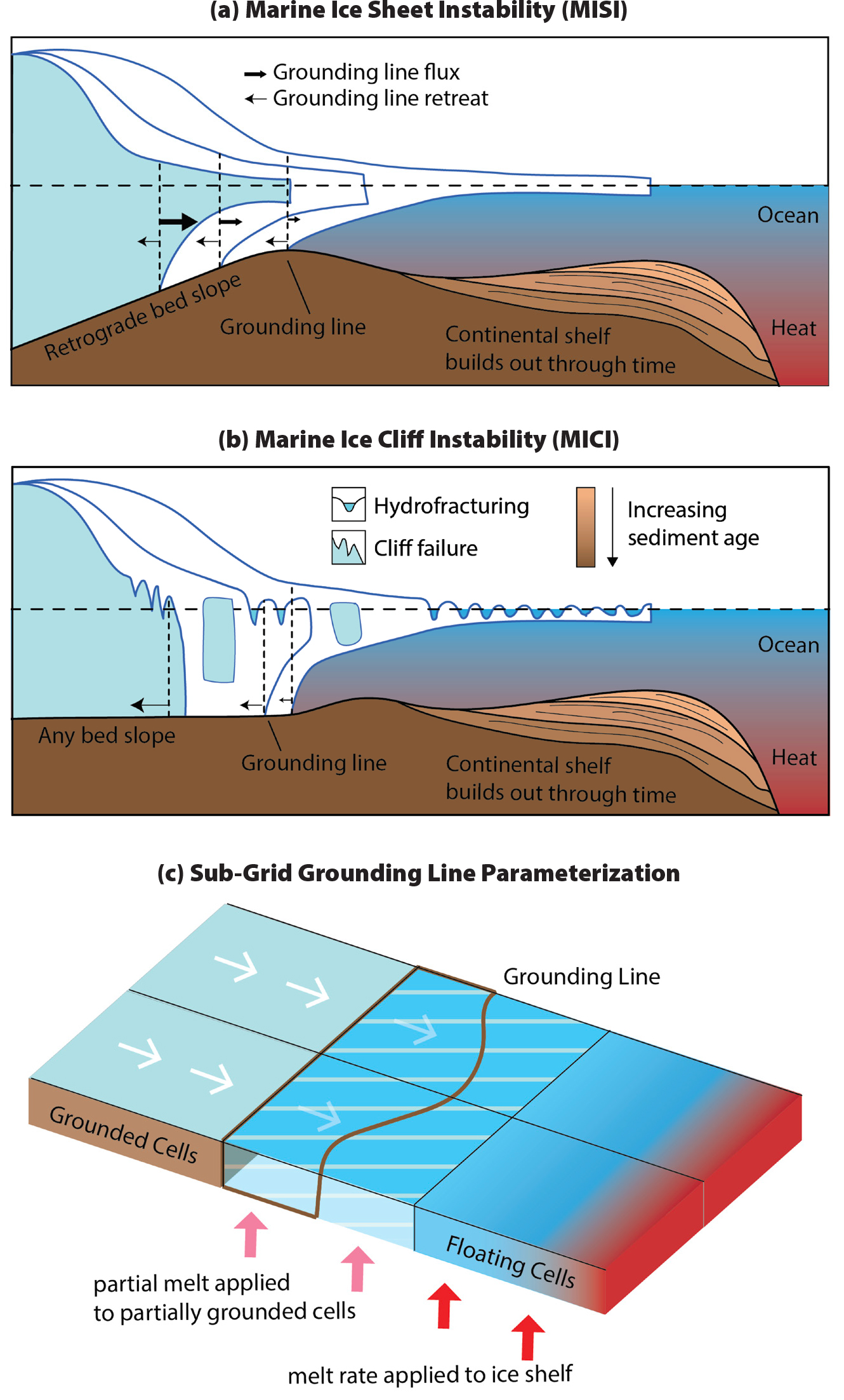
Figure 3. Schematics of processes discussed in the text. (a) Marine ice sheet instability on a retrograde slope. (b) Marine ice cliff instability (DeConto and Pollard, 2016). (c) The sub-grid grounding line melt parameterization (adapted from Seroussi and Morlighem, 2018). > High res figure
|
Similar to the problem of simulating retreat of the terrestrial Antarctic Ice Sheet, model simulations have largely failed to reproduce retreat in marine sectors of the ice sheet during the Pliocene. This is true for a range of ice sheet models (although they are all relatively low-resolution models with simplified physics) and climate model forcing (de Boer et al., 2015; Dolan et al., 2018). The only models that have successfully simulated retreat have required additional processes that enhance losses from ocean melting (Mengel et al., 2016; Golledge et al., 2017), surface melting (Hill et al., 2007), or calving (Pollard et al., 2015). We next review each of these approaches and their potential limitations.
Golledge et al. (2017) simulated retreat of the Antarctic Ice Sheet by ~9 m during the early Pliocene. This model includes a parameterization that affects ocean melting at the grounding line. The “sub-grid melt parameterization” applies a reduced ocean melt rate proportional to the fraction of model cells that are floating versus grounded (Figure 3c). This is controversial because it applies an ice shelf melt rate, albeit reduced, to all parts of the cell, including the grounded fraction that is upstream of the grounding line. This parameterization is used to overcome the limitations of using low-resolution ice sheet models in order to perform long-duration simulations that can be compared with paleoclimate data. It also builds on the increasing recognition that ice-ocean interactions occur over a broader grounding “zone” rather than a fixed grounding line. Similar sub-grid schemes are used to calculate the ice flux across the grounding line (Pollard and DeConto, 2009). However, tests of the sub-grid melt parameterization with higher-resolution models that are able to resolve the grounding line in more detail show that this scheme may overestimate mass loss from ice shelf melting (Seroussi and Morlighem, 2018). The sensitivity of ice sheet simulations to the inclusion of this parameterization can be large—the Antarctic Ice Sheet sea level projections for the emissions scenario RCP8.5 of Golledge et al. (2015) vary from 1.6 m to 3.0 m by 2300, with and without sub-grid melt.
Hill et al. (2007) forced an ice sheet model with constant climate forcing from a climate model run with an already partially retreated Antarctic Ice Sheet. This simulation resulted in retreat of the Antarctic Ice Sheet equivalent to ~9 m of sea level, driven by surface melting. This study is one example of a common approach to ice sheet modeling studies in which the ice sheet model and the climate model are not directly coupled. Therefore, the global surface topography, including the ice sheets, is prescribed in the climate model and large differences can develop between ice sheet extent in the ice sheet model and in the climate model. Any feedbacks from the retreat of the ice sheet are applied to the climate forcing before the ice sheet has retreated. There is therefore concern that the experimental design led to this result. Indeed, tests of alternative climate model forcing, without a collapsed ice sheet, do not produce a similar magnitude of retreat (Dolan et al., 2018). Approaches to forcing ice sheet models are evolving as more attention is paid to direct or asynchronous climate coupling to capture feedbacks between the ice sheets, the ocean, and the atmosphere (e.g., Golledge et al., 2019).
Pollard et al. (2015) introduced a scheme for the structural failure of large marine-terminating ice cliffs in combination with the hydrofracture of ice shelves. This scheme was based on earlier work of Bassis and Walker (2012) and is called “Marine Ice Cliff Instability” (MICI; DeConto and Pollard, 2016). Based on theoretical work, Pollard et al. (2015) introduced an upper limit for the height above water level that ice cliffs can reach. Beyond this limit, stresses exceed the strength of ice and there is brittle failure of the ice cliff. Ice cliffs can form when the buttressing ice shelves are rapidly removed. In the model, this is done using a scheme for hydrofracturing, which is caused by the rapid calving of ice shelves when meltwater and rain drain into surface crevasses. This process is similar to the disintegration of the Larsen B ice shelf, which occurred very rapidly (in ~1 month). Once an ice cliff fails, as long as the grounded ice is thick enough to keep failing, a runaway retreat can occur (Figure 3b).
There are many uncertainties associated with MICI, including the potential rate of ice cliff collapse, what is the failure threshold for subaerial ice cliffs, and whether ice shelves can be removed fast enough to generate sheer cliffs before ice flows into a more stable state (Bell et al., 2017; Clerc et al., 2019; Parizek et al., 2019; Robel and Banwell, 2019). Understanding the potential significance of MICI is limited by a lack of observations because there are only a few locations where MICI-like behavior occurs. Most Antarctic glaciers that have bedrock geometry favorable for MICI are currently protected by ice shelves, with the possible exception of Crane Glacier. In Greenland, the calving fronts of Helheim and Jakobshavn Isbræ Glaciers terminate with subaerial ice cliffs that reach ~100 m in height (Meredith et al., 2019). Observation of calving events at these glaciers is ongoing to improve understanding of MICI. However, these glaciers are situated in confined valleys and are not perfect analogues to the much wider calving fronts of Antarctica, such as that of Thwaites Glacier (Parizek et al., 2019). This active area of research has been stimulated in part by the rapid rates and high magnitudes of future sea level change in simulations that include these processes—up to ~8 m by 2300 under emission scenario RCP8.5 (DeConto and Pollard, 2016).
An alternative take on the Pliocene sea level problem is that retreat was confined to the marine sectors of the West Antarctic Ice Sheet. This scenario would be consistent with model predictions that do not include MICI (de Boer et al., 2015). When the uncertainties regarding Pliocene sea level estimates are interrogated more closely, this scenario is a possibility for at least some methods (Winnick and Caves, 2015; Gasson et al., 2016a). Indeed, Edwards et al. (2019) suggest that the simulations of DeConto and Pollard (2016) that do not include MICI and ice shelf hydrofracture are consistent with the lowest bounds on Pliocene sea level. The lack of consensus on the Pliocene sea level maximum means that this warm climate interval is currently of debated utility in discriminating between different ice sheet physics (Raymo et al., 2018). This situation was improved recently by the publication of two new studies on Pliocene sea level, one concerning overgrowths on speleothems measured in caves on Mallorca (Dumitru et al., 2019) and another the amplitude of glacial-interglacial sea level cycles based on grain-size analysis of cores drilled in New Zealand (Grant et al., 2019). Both of these support retreat of marine ice throughout Antarctica during the Pliocene. However, the uncertainties are still large and may not alter the conclusions of Edwards et al. (2019). Better constrained sea level estimates of the mid-Pliocene warm period remain critically important to resolving the debate over the stability of Antarctica’s marine ice.
Outlook and Conclusions
We have described the broad challenges that currently exist in simulating the response of the Antarctic Ice Sheet to climate changes in the geologic past. In sum, proxy records suggest greater ice sheet instability than is often captured by ice sheet modeling studies (Pollard and DeConto, 2005; de Boer et al., 2015). Maintaining a critical view of both data- and model-based histories of the Antarctic Ice Sheet is critical for connecting, and ultimately bridging the two related but often isolated disciplinary communities. We have focused on the separate challenges of simulating retreat of the “terrestrial” and “marine” sectors of the ice sheet and the different styles and mechanisms of ice sheet retreat during the warm intervals of the middle Miocene and the mid-Pliocene. These intervals were chosen as they arguably best characterize the model-data mismatch and provide clear examples of how new modeling approaches are probing longstanding mysteries.
Many other avenues not mentioned in this paper should be further explored by the next generation of ice sheet modelers. The role of opening and widening ocean gateways, in particular Drake Passage, in the inception, fluctuations, and persistence of ice on Antarctica remains debated (Figure 1; Kennett, 1977; Goldner et al. 2014). Coupling of ice sheet and solid Earth models demonstrates how and why different sectors of Antarctica may have become more or less prone to retreat through time (Austermann et al., 2015; Whitehouse et al., 2019). Ice sheets exert local influences on sea level and the surrounding ocean that can affect their stability, and these processes have only recently been included in ice sheet modeling studies (Golledge et al., 2019). Novel observations collected by marine mammals near calving fronts (e.g., Treasure et al., 2017) and autonomous vehicles under floating ice shelves (e.g., Spears et al., 2016) are challenging model parameterizations of the interactions between warm ocean water and the ice sheet. Such techniques will be critical for understanding active subglacial meltwater features (e.g., Drews et al., 2017) that may extend over a kilometers-wide “grounding zone” beneath the grounded ice sheet (e.g., Christianson et al., 2016). As observations become more detailed, we also find small-scale geological features that can be directly linked to ice sheet processes and thus offer tantalizing targets for models—for example, the observation of corrugation ridges in Pine Island Trough (Wise et al., 2017), “ladders and rungs” on the seafloor of the eastern Antarctic Peninsula (Dowdeswell et al., 2020), and paleo-meltwater channels in the Ross Sea region (Lewis et al., 2006; Simkins et al., 2017) and along the Sabrina Coast (Gulick et al., 2017). In these realms and others, the convergence of geologic data, new observations, and continental-scale models offers many productive paths forward for understanding past fluctuations of the Antarctic Ice Sheet. In further addressing the data-model inconsistencies highlighted in this paper, new developments in ice sheet models may reveal still more mysteries. Close collaboration between data- and model-focused communities will remain critical for moving forward.
ACKNOWLEDGMENTS
E.G. thanks the Royal Society and Natural Environment Research Council (NERC) grant “PLIOAMP” (NE/T007397/1) for financial support. B.K. is funded by a Lamont-Doherty Postdoctoral Fellowship. We thank Bas de Boer and guest editor Amelia Shevenell for comments that greatly improved the manuscript.