INTRODUCTION
Current scientific consensus identifies anthropogenic carbon emissions as the major cause of the unprecedented rates of atmospheric and oceanic warming observed over the last few decades (IPCC, 2014). Between 1971 and 2010, the upper 75 m of the ocean warmed by 0.11°C per decade, while snow and ice cover concurrently diminished (IPCC, 2014). Such trends are predicted to continue throughout the rest of this century. By the year 2100, sea surface temperatures are expected to warm by 1.1°–2.9°C based on the most conservative B1 emission scenario, and by 2.0°–5.4°C based on the more realistic A2 scenario (IPCC, 2007), with warming continuing beyond 2100 under all predicted scenarios (IPCC, 2014). This warming is considered irreversible on a multicentury timescale unless efforts are made to actively remove CO2 from the atmosphere (IPCC, 2014).
The impacts of these changes are already apparent in many ecosystems, but perhaps most markedly in marine environments, particularly through increased durations of high-temperature events. The 2016 mass bleaching of corals on the Australian Great Barrier Reef, for example, resulted from the cumulative impacts of several anomalously warm months (Hughes et al., 2017). Such cumulative effects are expected to manifest themselves as changes in many aspects of marine ecosystems, including total biomass, community structure, diversity, and species interactions—as well as changing host-pathogen dynamics. Here, we explore the cumulative impacts of temperature changes on host-pathogen dynamics, using examples from the poles to the equator.
The persistent trends in environmental variables driven by global climate change motivate scientists to quantify the effects of environmental and physiological changes on host organisms and pathogens (e.g., Maynard et al., 2016). Changes in the environment can result in outcomes that favor either host or pathogen, increasing or decreasing local infection rates (Danovaro et al., 2011; Burge et al., 2014). Hosts may exhibit impaired immune response, habitat loss, or range expansion, while pathogens may develop increased virulence, pathogenicity, expanded ranges, or decreased range overlap with their hosts (Harvell et al., 2002). Better understanding of these dynamics will improve our ability to predict changes in host-pathogen interactions and the expected impacts on ecosystem structure and composition. At an ecosystem scale, these impacts can cascade through trophic pathways, affecting organisms from primary producers to top predators.
Changes in temperature, pH, O2, sea ice extent, and many other environmental variables contribute to the impact of climate change on disease in marine systems. Here, we use temperature and sea ice cover as the primary variables through which we explore changes in host-pathogen relationships. Temperature accounts for much of the spatial and temporal biogeographic structure of the ocean (Kordas et al., 2011) through its effects on the growth, survival, health, and reproduction of marine organisms (Doney et al., 2012). Our decision to use temperature as an indicator variable was also governed by the availability of measured temperature responses for many taxa (both hosts and pathogens alike) and global model temperature projections for the next hundred years.
Temperature is also a particularly useful proxy for global climate change. Furthermore, many hosts display cumulative responses to temperature trends. For example, cumulative heat stress—rather than any single daily temperature—is a primary driver of bleaching for many corals (Berkelmans 2002; Heron et al., 2016; Hughes et al., 2017). We use output from global climate models to explore the effects of climate change on selected host-pathogen systems. We quantify changes in the annual cumulative duration of sea surface temperatures and sea ice concentrations relative to thresholds relevant to particular host-pathogen interactions. Using case studies as examples, we explore four fundamental modes through which climate change is likely to affect host-pathogen dynamics.
- Increased Host Stress. Many marine taxa exhibit stress in response to changes in their physical environments (Burge et al., 2014). Small increases in global ocean temperature could lead to broad-scale changes in stress-induced host immune responses (Harvell et al., 2002). With their global distributions, sea urchins and their pathogens serve as useful models for this response (Maes and Jangoux, 1984; Lester et al., 2007; Becker et al., 2008).
- Increased Pathogen Virulence. Elevated temperatures increase the virulence of many marine pathogens (Burge et al., 2014) by increasing pathogen metabolism and fitness, ultimately leading to higher rates of transmission (Karvonen et al., 2010). The pathogen Vibrio coralliilyticus and one of its coral hosts, Pocillopora damicornis, provide a useful case study of increased virulence in the face of warming ocean temperatures driven by anthropogenic climate change. Vibrio species are globally distributed and can infect a wide range of organisms. The increasing success of Vibrio in a changing ocean may have important implications for marine community composition (Ben-Haim et al., 2003a; Kimes et al., 2012; Vezzulli et al., 2013).
- Pathogen Range Expansion. Regionally, warming seas may allow pathogens to expand farther into host habitat (de Montaudouin et al., 2010; Xu et al., 2017). The well-studied interaction between the parasite Perkinsus marinus and its host, the oyster Crassostrea virginica, is a useful example of this dynamic. This system is representative of host species whose ranges currently extend beyond the ranges of their pathogens; with increasing temperatures, pathogen ranges are likely to encroach into those of unaffected populations.
- Host Range Changes. Shifts in species distributions as a result of altered habitat may increase exposure rates and transmission of certain pathogens, or introduce novel pathogens into a system. This can be seen in the interactions between Brucella spp. and their marine mammal hosts, such as pinnipeds. Temperatures in polar regions, where most pinniped species are found, are increasing at nearly twice the global average rate, resulting in substantial reductions in ice cover (IPCC, 2007; Kovacs, 2011). These changes will manifest in different outcomes for species that depend upon sea ice versus species that avoid it. Range restriction of ice-dependent species has the potential to increase local population densities, which is likely to favor increased transmission of pathogens within populations. Simultaneously, range expansion of species that avoid polar ice may facilitate range expansion of their pathogens, leading to increased transmission among populations.
METHODS
Climate projections used here were obtained from A2 scenario data made available by the Intergovernmental Panel on Climate Change (IPCC) Fourth Assessment SRES AR4 CMIP3 experiments (Meehl et al., 2007). The data are freely available from the IPCC Data Distribution Center (http://www.ipcc-data.org). The A2 scenario emphasizes economic and global integration over environmental or regional solutions. It is characterized by a more divided world (i.e., more independently operating, self-reliant nations), continuously increasing population, and slower economic and technological development compared to other scenarios (IPCC, 2007). The A2 scenario projects a best-estimate global surface temperature rise of 3.4°C with a likely range of 2.0°C to 5.4°C by 2100. Models for sea surface temperature and sea ice coverage were selected based on their availability and ease of online access. Monthly sea surface temperature projections were generated by the ECHAM5/MPI-OM model from the Max-Planck Institute for Meteorology (Hamburg, Germany). The model had a spatial resolution of 1.9° × 1.9° and is described in detail by Roeckner et al. (2004). The ECHAM5/MPI-OM model from 2000 to 2100 projects a global sea surface temperature rise of 2.5°C (Figure 1).
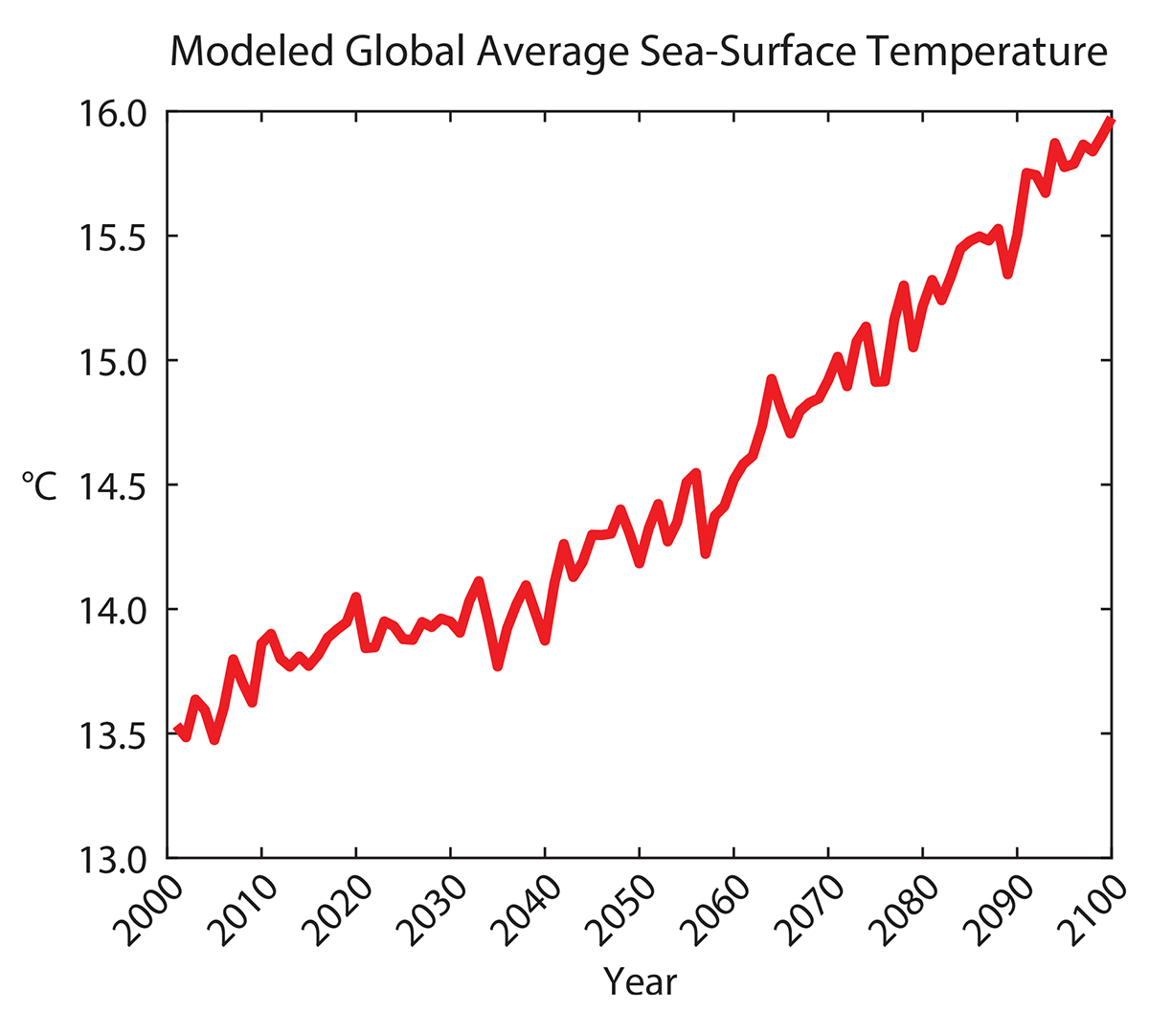
Figure 1. Projected sea surface temperatures from 2000 to 2100 from the ECHAM5/MPI-OM model using the A2 climate scenario (Meehl et al., 2007). > High res figure
|
Using the ECHAM5/MPI-OM model, average sea surface temperatures from 2001 to 2017 were calculated to use as a baseline (Figure 2a–c) against projected sea surface temperatures in 2050 and 2100 (Figure 2d–i), averaged over a five-year span to account for natural annual variability. From these data, we calculated the number of months each year during which temperature was greater than a given threshold: 24°C, 27°C, or 30°C. We then subtracted the baseline (2001–2017) from the projected number of months exceeding the temperature thresholds. These yearly anomalies were used to make predictions concerning the effects of global climate change on various aspects of host-pathogen relationships in different regions. All plots were made in MATLAB (version 2016b) using m_map (version 1.4b).
Monthly sea ice coverage projections were obtained from the CM2.0 model of the Geophysical Fluid Dynamics Laboratory (GFDL; Princeton, NJ, USA). The GFDL CM2.0 model has an ocean resolution of 0.3°–1.0° × 1.0° with depth as the z-coordinate and an explicit free surface top boundary condition. Gnanadesikan et al. (2006) provide a detailed description of the model, including benefits and drawbacks of the model parameters. Ice area concentrations of 75% and 90% are used to compare seasonal changes in ice area for 2017, 2050, and 2100, and mapped according to periods of known haul-outs for ringed (March–May) and fur (June–July) seals.
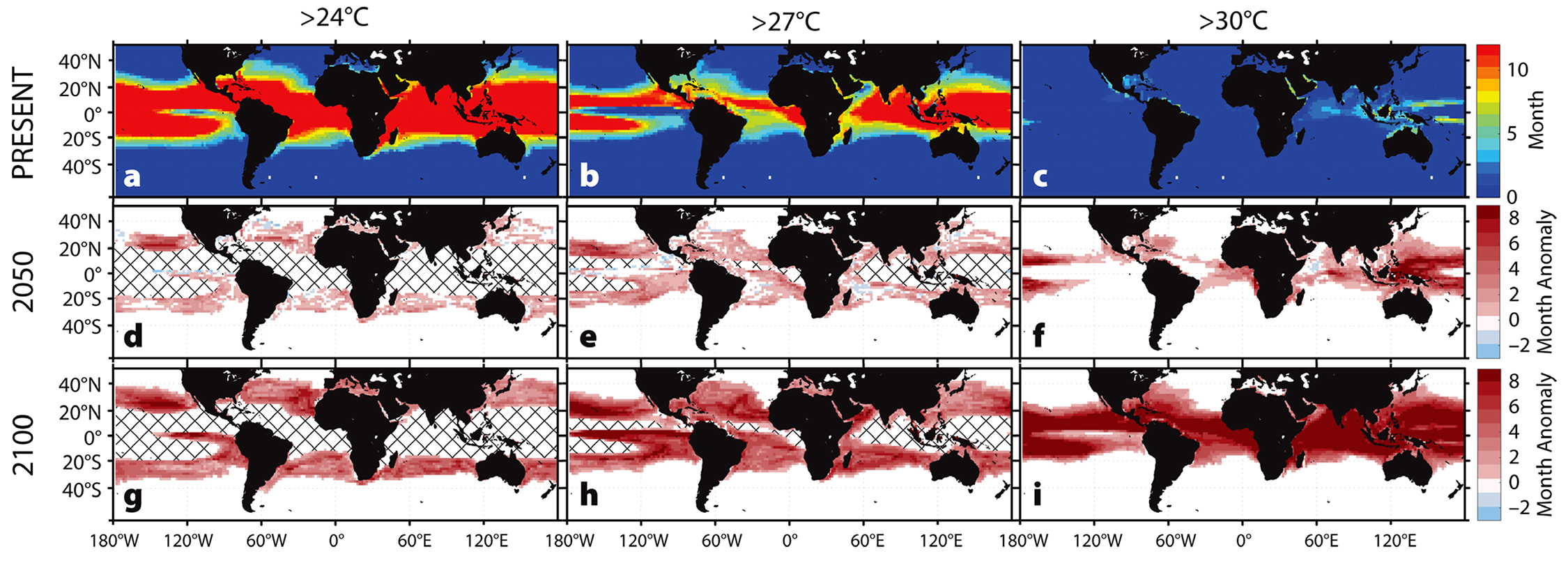
Figure 2. Predicted increases in the duration of warm-water events. (a–c) Average number of months sea surface temperatures exceeded the given temperature threshold (Present: 2001–2017). The baseline durations (a–c) were subtracted from projected durations in 2050 (d–f) and 2100 (g–i) to give predicted annual anomalies in the duration of warm-water events. Cross-hatching denotes areas where all 12 months exceed the threshold temperature duration of the baseline. > High res figure
|
CASE STUDIES
Increased Stress Inhibits Host Recovery
Urchins, present in all ocean basins and occupying a variety of temperature ranges, may represent an indicator for increases in host infections due to thermal stress-induced declines in immune responses. Bald sea urchin disease is an umbrella term describing an assortment of urchin diseases that trigger similar symptoms and that are caused by a variety of pathogenic bacteria (Jangoux, 1987; Lester et al., 2007; Becker et al., 2008). The bacteria consume healthy urchin tissue to form distinct lesions that range in size from a few millimeters to more than one-third of the host’s surface, with many urchins afflicted by multiple lesions (Maes and Jangoux, 1984; Jangoux, 1990; reviewed by Becker et al., 2008). Regeneration and recovery from bald sea urchin disease is possible; however, in cases where the skeleton is breached or a majority of the urchin is covered in lesions, death is common (Maes and Jangoux, 1984; Jangoux, 1987, 1990; reviewed by Becker et al., 2008).
Infection rates for bald sea urchin disease in both tank experiments and multiple real-world mass mortality events are strongly correlated with increases in temperature (Scheibling and Stephenson, 1984; Ward and Lafferty, 2004; Girard et al., 2012; Brothers et al., 2016; Sweet et al., 2016). At higher temperatures, urchins experience thermal stress, which initially triggers a heightened immune response (Brothers et al., 2016). Despite these immunological responses, increased infection rates often occur when elevated temperatures persist or are more extreme. Furthermore, urchins often exhibit decreased activity and feeding during extreme heat events, as well as decreased fecundity, which can restrict recovery of individuals and populations (Farmanfarmaian and Giese, 1963; Lester et al., 2007). While negatively impacting the urchins, these extreme heat events appear to favor the pathogens, increasing growth and transmission rates, as well as improving bacterial survivorship (Brothers et al., 2016; Sweet et al., 2016). The longer the urchins remain in a state of stress, the higher their susceptibility to disease (Brothers et al., 2016; Sweet et al., 2016). Though recovery is possible, urchin species impacted by a bald sea urchin disease event tend to exhibit increased mortality in persistently elevated seawater temperatures (Lester et al., 2007; Girard et al., 2012; Brothers et al., 2016).
Critical temperatures above which host immune responses decrease are 23°C for Strongylocentrotus purpuratus, Heliocidaris erythrogramma, and Strongylocentrotus intermedius (Farmanfarmaian and Giese, 1963; Agatsuma, 2007; Brothers et al., 2016) and 29°C for Paracentrotus lividus (Boudouresque and Verlaque, 2007). We predict that the number of months exceeding each species’ critical temperature will increase from the baseline through 2100 (Figure 3). These increases will grow spatially and become longer in duration over the next 100 years in both temperate and subtropical regions.
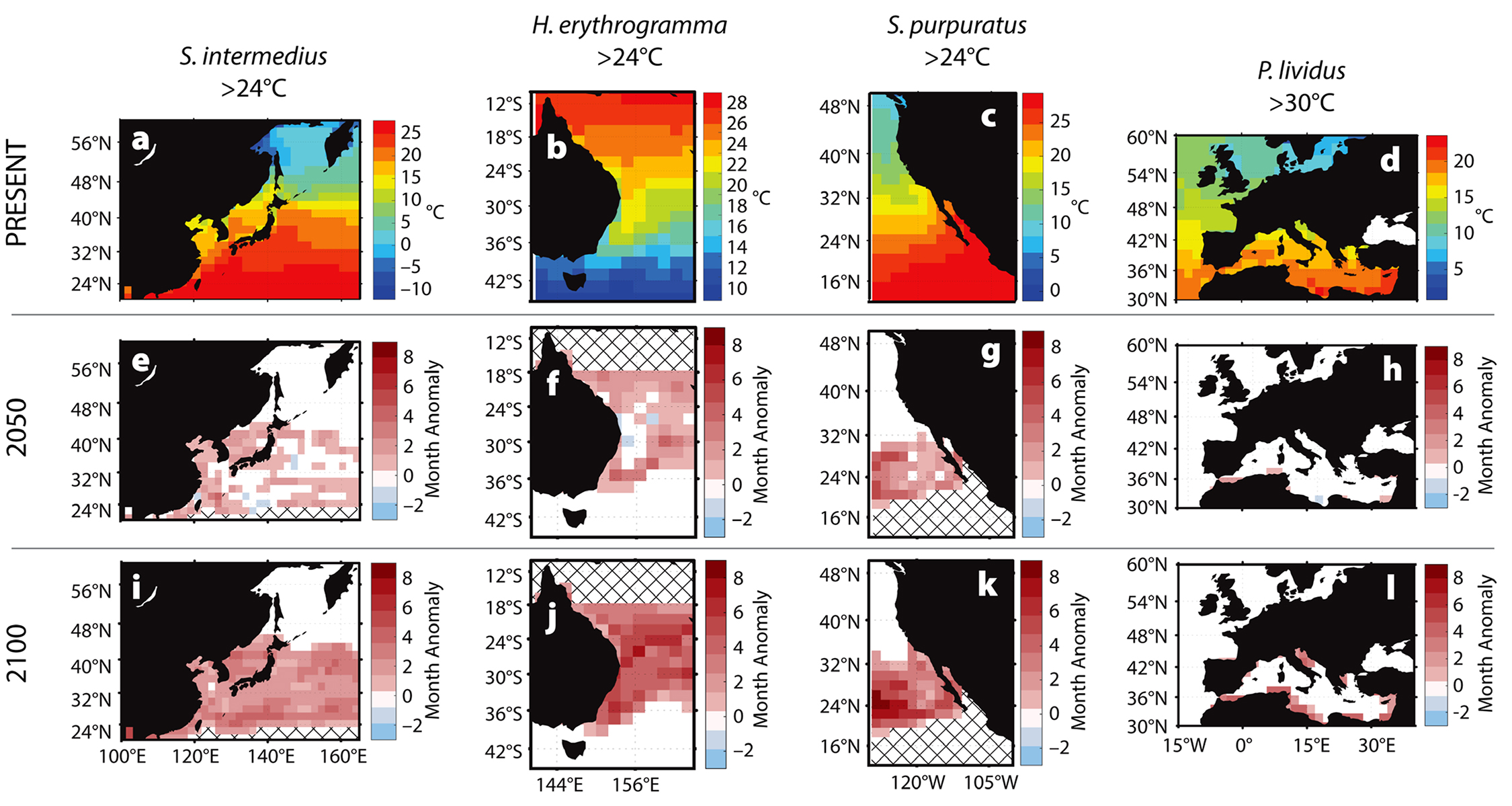
Figure 3. Predicted increases in the duration of warm-water events for four species of urchin from four geographic areas affected by bald sea urchin disease. (a–d) Baseline temperature conditions (2001–2017). (e–h) Changes in the number of months the temperature is predicted to exceed the given threshold by 2050, and (i–l) by 2100. Cross-hatching same as Figure 2. > High res figure
|
Predicted increases in the frequency, duration, and intensity of warm-water events suggest reduced survival and recovery from future mass mortality events for many urchin populations. For example, two to four more months of above-critical temperatures each year in the Mediterranean Sea will affect P. lividus by 2100 (Figure 3d,h,l). In coastal regions, up to five additional months of temperatures above 30°C are predicted, a trend that may lead to local extinctions. Similarly, S. intermedius may be exposed annually to two more months of above-critical temperatures around Japan and the Korean peninsula by 2100 (Figure 3a,e,i). In the global warming hotspot off the east coast of Australia, home to H. erythrogramma, at least four to six more months of above-critical temperatures are predicted by 2100, with some regions expected to increase by seven or eight more months (Figure 3b,f,j). The most marked temperature increases occur in the range of S. purpuratus along the west coast of North America, with seven to eight more months of elevated temperatures predicted for some areas each year (Figure 3c,g,k). The region extending across the Pacific between 16°N and 36°N may exceed 27°C for eight additional months per year, with potentially extreme consequences for resident populations (Figure 2).
These predicted increases in the frequency, duration, and intensity of warming events will cause urchins—and many other organisms living within these areas—to respond to enhanced thermal stress with altered immune responses and increased mortality rates due to pathogenic infection.
Increased Temperature Increases Pathogen Virulence
Vibrio are among the most common pathogens in the marine environment (Vezzulli et al., 2013) and comprise the largest genus (81 species) in the Vibrionaceae family (Thompson et al., 2007). In corals alone, geographically distinct strains of V. coralliilyticus can cause specific infections and diseases based on host, location, and local temperature (Pollock et al., 2010).
Pocillopora damicornis is found throughout the Pacific and Indian Oceans and in some areas serves as the dominant reef engineer (Combosch and Vollmer, 2011; Hoeksema et al., 2014). Most scleractinian corals rely for a significant portion of their energy requirements on intracellular, symbiotic, photosynthetic dinoflagellates (“zooxanthellae” primarily of the genus Symbiodinium; Bourne and Munn, 2005; Bourne et al., 2009). V. coralliilyticus is known to infect P. damicornis, impacting both the coral host and its zooxanthellae (Ben-Haim et al., 2003b).
The host-pathogen interactions of P. damicornis and V. coralliilyticus are critically dependent on seawater temperature. At 25°C, V. coralliilyticus becomes infectious to P. damicornis (Ben-Haim et al., 2003b). Above 27°C, pathogen virulence increases significantly, coral fitness is reduced, and P. damicornis is no longer able to adequately resist infection. In addition, multiple virulence factors, including transcriptional regulation, quorum sensing, antimicrobial resistance, and toxin secretion systems, are upregulated at this temperature (Kimes et al., 2012). Extracellular proteases produced by V. coralliilyticus can cause subsequent lysis and mortality of P. damicornis (Ben-Haim et al., 2003a), which, in laboratory studies, occurs within two weeks at temperatures above 27°C (Ben-Haim et al., 2003b).
The tropics (23.5°S to 23.5°N) already exhibit consistent annual temperatures of 24°C to 27°C (Figure 2a,b), and that range will expand into the future. Heat-stressed corals and their symbionts release higher concentrations of dimethylsulfoniopropionate (DMSP), which acts as a trigger for chemotaxis (directional movement in response to a chemical stimulus) and chemokinesis (a change in swimming behavior in response to a change in chemical concentration) in V. coralliilyticus (Garren et al., 2014, 2016). Upon entry to the host cells, V. coralliilyticus targets the P. damicornis zooxanthellae (Ben-Haim et al., 2003a), resulting in the expulsion of the symbionts and consequent bleaching (Dove and Hoegh-Guldberg, 2006). Recovery may be possible if temperatures return to an acceptable level for a long enough period. However, with projected increases in sea surface temperatures for 2050 (Figure 2d–f) and 2100 (Figure 2g–i), mass coral mortalities can be expected (D’Croz and Maté, 2004).
With elevated sea surface temperatures, we can expect to observe shifts in the dynamics of V. coralliilyticus and P. damicornis (Ben-Haim et al., 2003a,b). At temperatures greater than 30°C, V. coralliilyticus abundance increases by up to four orders of magnitude relative to 24°C, further increasing the likelihood of infection (Tout et al., 2015). Climate models predict that by 2050, a portion of the Indo-Pacific will experience up to seven additional months of temperatures greater than 30°C (Figure 4b), and by 2100 at least eight additional months of temperatures greater than 30°C annually for the entire region (Figure 4c).
Most of the tropics are already near or above these threshold temperatures for the majority of the year (Figure 2), and these conditions are expected to expand poleward. As a result, the spatial extent of certain highly virulent pathogens may expand as climate change progresses.
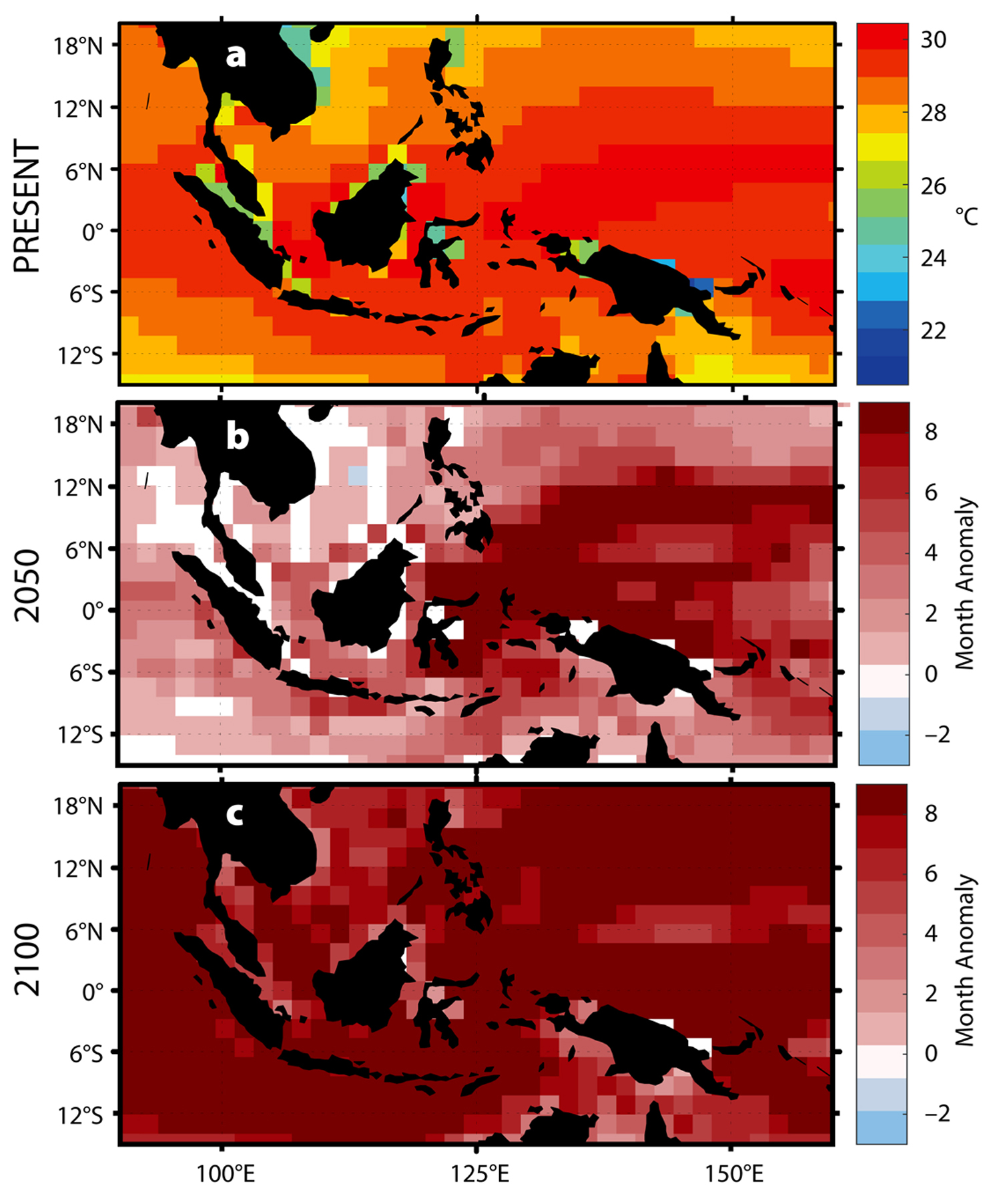
Figure 4. Predicted changes in the thermal habitat of V. coralliilyticus. (a) Present annual average temperatures. (b–c) Predicted changes in the duration of warm-water events (>30°C) in (b) 2050 and (c) 2100. > High res figure
|
Temperature Facilitates Pathogen Range Expansion
While the virulence of local pathogen populations is likely to increase, we also expect to see pathogen range expansion in response to increasing temperatures. To explore this possibility, we considered the single-celled protozoan Perkinsus marinus and its economically important eastern oyster host Crassostrea virginica. Oysters are often used as indicators of environmental health—their efficient filter-feeding mechanisms increase the exposure of their tissues to pathogens in the surrounding waters (Segarra et al., 2010; Jenkins et al., 2013; Bidegain et al., 2016). Oysters are also globally distributed, with a wide range of temperature tolerances (Soudant et al., 2013); the spread of P. marinus to C. virginica has historically been restricted by the temperature threshold of the pathogen.
Originally classified as the fungus Dermocystidium marinum, which led to the disease name “dermo” (Bower et al., 1994), P. marinus infects several mollusc species but has been most extensively studied in C. virginica (Bower, 2013). P. marinus competes with its oyster host for nutrients and is known to cause disease and mortality (Bower et al., 1994; Soudant et al., 2013). The infected oyster becomes stressed, tissues pale in color, and gamete production and growth slow. Emaciated, the mantle shrivels and pulls away from the shell, and pockets of pus-like fluid develop (Bower et al., 1994). Lysis of tissues and blockage of blood vessels cause fatality, though many oysters can persist up to three years with active infections (Sunila, 2007). Adult oysters can tolerate sustained temperatures of 2°C to 36°C, and up to 49°C for short intervals (Banks et al., 2007), with the optimal range between 20°C and 30°C. This wide temperature tolerance allows oysters to inhabit the majority of the world’s coastlines (Banks et al., 2007). The temperature tolerance of P. marinus overlaps that of C. virginica, with growth between 18°C and 20°C, increase in infection intensity above 20°C, and highest infection intensity as temperatures near 25°C (La Peyre et al., 2008). P. marinus can survive down to 4°C (La Peyre et al., 2008).
Along the east coast of the United States, our analyses predict longer periods of sustained elevated temperatures, which may allow the range of P. marinus to expand into the established habitat of C. virginica and increase the potential for infection (Figure 5). Annual durations of ocean temperatures greater than 24°C are predicted to increase up to four months by 2050, and up to six months by 2100, in this region. The long duration of elevated temperatures creates the potential for pathogens to become established farther north along the coast. This potential for pathogen range expansion is corroborated by changes during the 1990–1992 warming period when sea surface temperatures increased by 0.27°C to 0.77°C per year along the US east coast (Cook et al., 1998). The subsequent P. marinus expansion devastated C. virginica populations, causing damage to previously unaffected oyster populations. Some populations suffered infection rates of 90%–100% of individuals, with overall mortality reaching 60%–80% (Ford and Smolowitz, 2007).
Range expansions of pathogens into new areas where hosts may be unaccustomed to infection can thus result in unusually high mortality events. As these range shifts transition from episodic to permanent, relationships between hosts and their pathogens are likely to change.
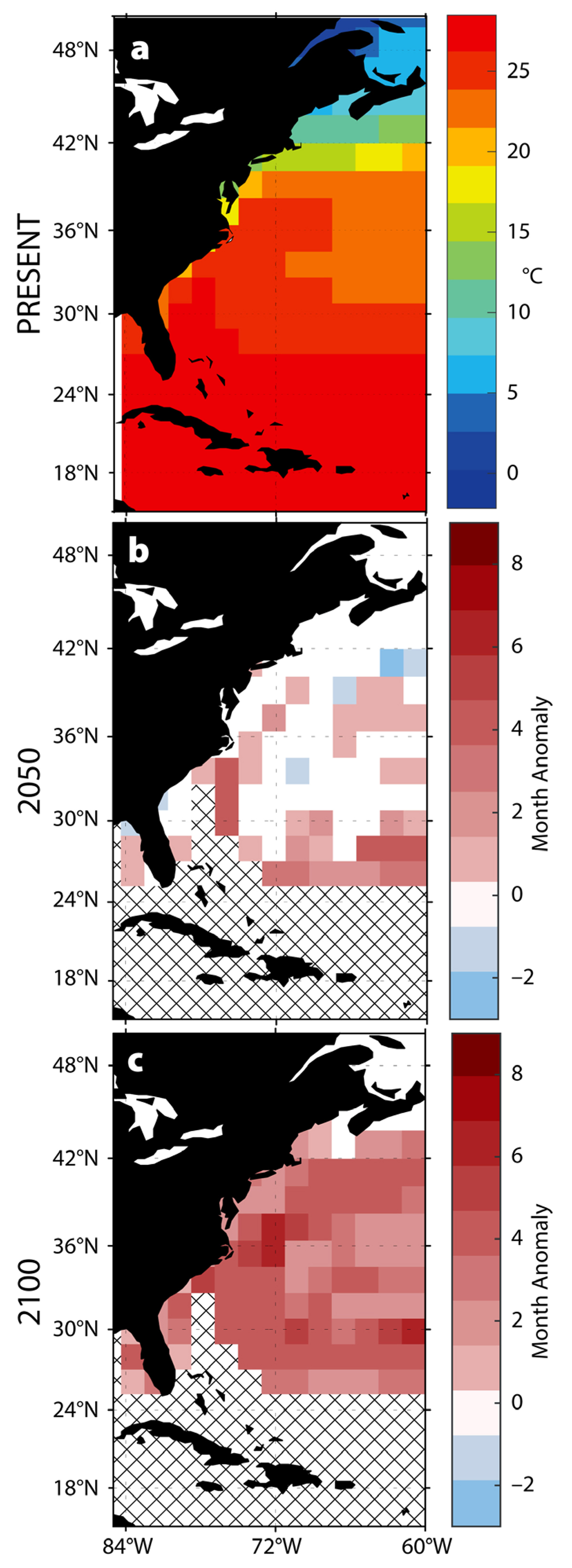
Figure 5. Predicted changes in the thermal habitat of P. marinus. (a) Present annual average temperatures. (b–c) Predicted changes in the duration of warm-water events (>24°C) in (b) 2050 and (c) 2100. > High res figure
|
Biogeographic Shifts Increase Incidence of Infection
Due to the disproportionately higher rate of temperature change observed at the poles, organisms such as pinnipeds may exhibit substantial biogeographic shifts in response to changes in suitable habitat availability. Pinnipeds are widely distributed throughout Arctic and Antarctic waters, with some species extending far into the subtropics (Pompa et al., 2011). Because pinnipeds must haul out on land or ice for breeding and pupping, they are at risk of exposure to both marine and terrestrial pathogens. Brucella, the causative agent of brucellosis, is a genus of gram-negative coccobacillus bacteria found in both marine and terrestrial environments. Brucella grows between 20°C and 40°C and optimally at 34°C (de Figueiredo et al., 2015). Brucella species do not multiply outside their hosts, but can persist for months in 10°C to 15°C conditions and for years in frozen tissue (Nymo et al., 2011). Scholz et al. (2008) demonstrated that one species, B. microti, has also found a long-term reservoir in soil. Because this wide temperature range enables Brucella to survive throughout the world ocean, instead of considering the direct impacts of Arctic temperature change on Brucella survival, we focused on changes in intra- and inter-population transmission rates as a result of sea ice loss.
Ten species of Brucella are recognized, with B. pinnipedialis and B. ceti primarily affecting their eponymous marine mammal groups. High Brucella seroprevalence rates have been observed among several pinniped species, such as hooded seals (Cystophora cristata) in the North Atlantic and Barents Sea (35%–38%), and common seals (Phoca vitulina) along the coast of Scotland (49%; Spickler, 2009). Brucellosis can present a wide variety of clinical symptoms: infection of reproductive tissue resulting in placentitis and spontaneous abortion; neurobrucellosis, associated with tremors, seizures, and strandings; and damage to tissue and joints as well as respiratory problems caused by systemic brucellosis (Thakur, 2012). Brucella spp. have also been found in the tissues of apparently healthy individuals (Spickler, 2009).
There have been multiple reported cases of Brucella infecting species other than their preferred hosts, and laboratory studies suggest that B. pinnipedialis has the potential to infect a wider range of animals (Lambourn et al., 2013). Vertical transmission is thought to occur during gestation and lactation, while horizontal transmission may take place via contact with infected individuals or their aborted fetuses, placentas, birth fluids, and secretions (Thakur et al., 2012).
Diminishing sea ice cover in the Arctic may increase occurrences of brucellosis among pinnipeds by increasing local population densities of pagophilic (ice-dependent) seals. Most of these species currently haul out on ice in low densities, generally fewer than one to two animals per square kilometer (Young et al., 2015). Using predicted Arctic ice cover during the pupping season from the GFDL CM2.0 model, we looked at changes in reproductive habitat for ice-dependent species (Figure 6). Based on our predictions, substantial reductions in ice and snow available for excavating subnivean birth dens are likely to increase competition for prime whelping, breeding, and molting sites among pagophilic species. Greater densities and increased contact among reproductive females may increase transmission of Brucella. For species that almost never haul out on land and prefer habitat with 75%–90% ice coverage (e.g., bearded seals, Erignathus barbatus, and ringed seals, Phoca hispida), this habitat constriction is particularly apparent (Figure 6; Simpkins et al., 2003).
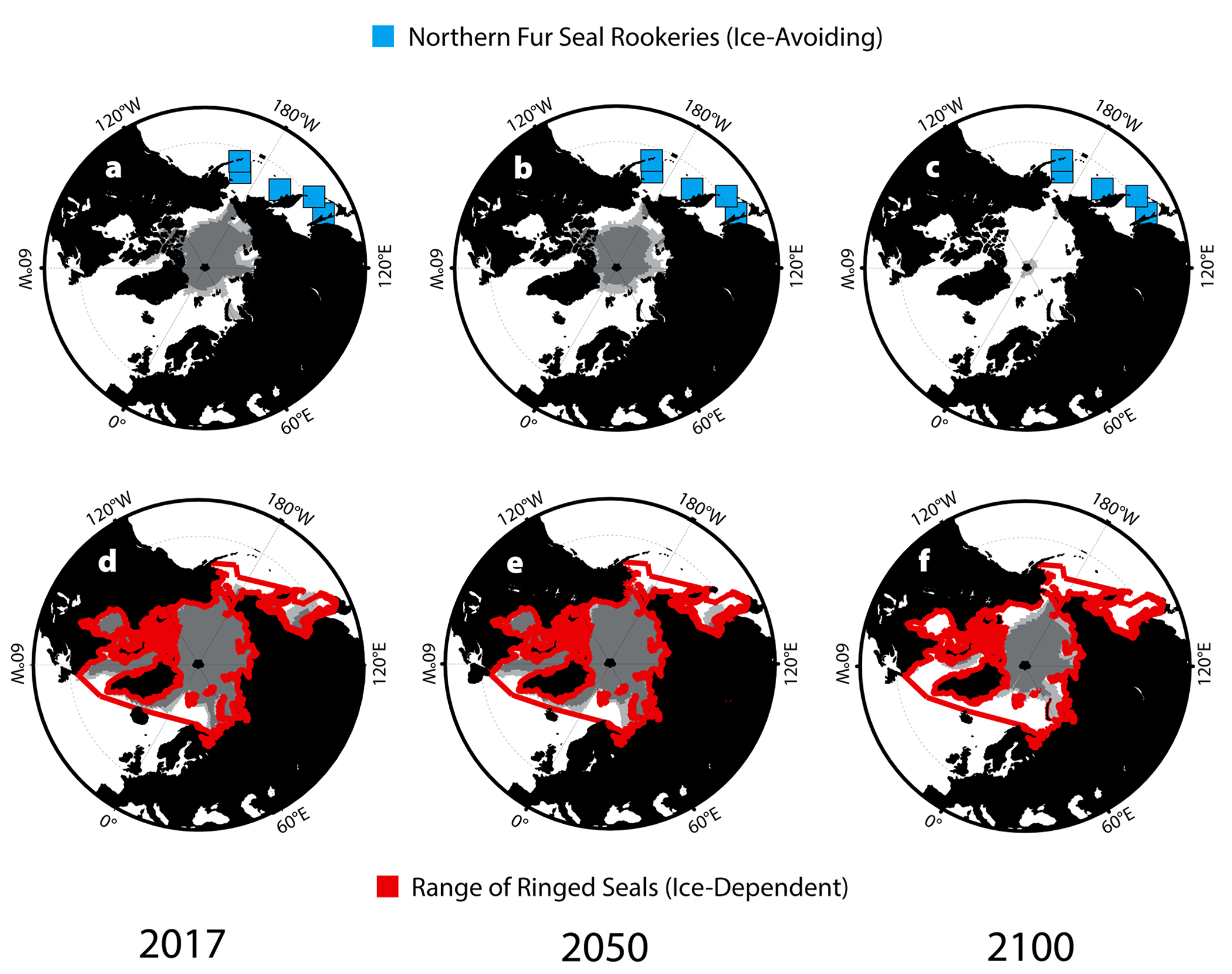
Figure 6. Habitat ranges for pagophilic and land-breeding species throughout the North Pacific with 75% and 90% ice cover (light and dark gray, respectively) for 2017, 2050, and 2100. (a–c) Island rookeries for the northern fur seal (Callorhinus ursinus) from June–July. (d–f) Peak habitat range for ringed seals (Phoca hispida) from March to May. > High res figure
|
Brucellosis may also spread following range expansion of land-reproducing pinnipeds that exploit exposed coastlines for their high-density breeding colonies. The northern fur seal (Callorhinus ursinus) currently ranges throughout the North Pacific, with five well-established rookeries on islands spanning the Okhotsk and Bering Seas (Figure 6). Retreating ice is expanding the Arctic passage between the North Pacific and the North Atlantic during the summer months, allowing access to new foraging grounds for many non-ice-dependent species (Figure 6; Kovacs et al., 2011). Such range expansion can result in contact among previously separated populations of marine and terrestrial Brucella-carrying species, contact with existing soil reservoirs of Brucella, establishment of new soil reservoirs of Brucella, and possible future transmission to other formerly isolated populations. As fur seal foraging grounds expand, there is also potential for the establishment of new rookeries exploiting this new territory. The breeding colony at Bogoslof Island, a volcanic island formed in the Bering Sea during the nineteenth century, was established circa 1980 and has since grown rapidly (Towell and Ream, 2015).
Seals that haul out on ice and land in different parts of their ranges or during different seasons (e.g., gray seals, spotted seals; Figure 6) exhibit reproductive behaviors and population densities that are somewhere between those of the strictly pagophilic and the land-breeding species. This may expose these species to a combination of the risks outlined above for both ice- and land-pupping pinnipeds.
Variations in ocean temperature and sea ice coverage associated with climate change will alter distributions of marine organisms, both expanding and contracting ranges. These changes in biogeography will alter pathogen transmission within and among populations and species.
DISCUSSION
As climate change continues to alter the natural world, species must adapt to survive or be faced with extinction. Average surface temperature is projected to increase by 2°C to 5.4°C within the next century (IPCC, 2007), and our analyses show increasing geographic extent and annual durations of above-critical temperatures. While there exists great variability in host-pathogen interactions, we chose case studies to illustrate some potential effects of increased heat-event durations on host-pathogen dynamics. In particular, we explored:
- Increased Host Stress. From our analyses, we predict that extended durations of above-critical temperatures will increase host stress levels and susceptibility to pathogens, potentially leading to local extinctions. We speculate that many marine organisms will become more susceptible to infection with global climate change, as illustrated by the case study of bald sea urchin disease.
- Increased Pathogen Virulence. While establishing direct causation between climate change and pathogen virulence may be difficult, cumulative climate change impacts are likely to increase the virulence of many marine pathogens by influencing factors such as metabolism, growth rate, and migration. Furthermore, sustained elevated temperatures could extend the length of the transmission season, ultimately leading to an increase in the frequency, duration, geographic extent, and intensity of infections worldwide. The temperature-dependent dynamic of V. coralliilyticus infection of P. damicornis provides an example of these potential changes.
- Pathogen Range Expansion. Range expansions are predicted to become more frequent with increasing sea surface temperatures (Figures 1 and 2). These changes will be particularly evident in the subtropical and temperate bands between 23.5° and 40° latitude, where significant increases in the number of warm months each year will give temperature-constrained pathogens considerable freedom to expand their ranges poleward (Figure 5). This will put unaffected populations at risk, as we expect to see with P. marinus infecting previously dermo-free populations of C. virginica.
- Host Range Changes. Host habitat constriction is likely to increase population densities and thus accelerate rates of intraspecies infection. Habitat expansion, on the other hand, is likely to cause novel contact among populations and disease vectors or reservoirs, potentially increasing interspecies infection. Both of these outcomes have the capacity to increase the incidence and geographic extent of infection by pathogens in changing physical environments, and can be explored through Brucella infection of various Arctic pinnipeds.
Our case studies illustrate how increasing temperatures will have global impacts on host-pathogen relationships, with regionally differentiated outcomes. By 2100, we expect increases of four to eight months of unusually high temperatures in temperate regions, largely due to the predicted migration of temperate zones poleward (Figure 2). Organisms with restricted dispersal (e.g., urchins and oysters) will have difficulty acclimating to temperature changes (Figures 3 and 5). In polar regions, sea ice melt may drastically constrict suitable habitats (Figure 6), while in the tropics, average monthly temperatures (>30°C) may become too high for even warm-water corals (Figure 4). Furthermore, pathogens currently restricted to narrow temperature bands may expand to create greater geographic overlap with host habitats (Figure 5).
Though we have focused on temperature as the dominant climate change forcing due to its ubiquitous impact on coastal and Arctic marine environments and the availability of literature on the subject, temperature will not act in isolation on marine communities: anthropogenic climate change is also causing our ocean to acidify, stratify, and exhibit increased hypoxia. In coastal oceans, there are shifts in freshwater input and sedimentation as a result of changing land-use and precipitation patterns. Moreover, humans are significantly impacting biodiversity through industrial fishing and aquaculture practices (IPCC, 2014).
“Changes in temperature, pH, O2, sea ice extent, and many other environmental variables contribute to the impact of climate change on disease in marine systems.”
Not all changes are predicted to have negative consequences for the hosts, and some outcomes may be mixed (Danovaro et al., 2011). Pathogens may be less tolerant of temperature changes than their hosts, resulting in decreased pathogen abundance and local incidence of infection (Holt et al., 1989; Harvell et al., 2002). Changes in host abundance and distribution may also decrease infection rates by disrupting complex pathogen life cycles, which can rely on multiple hosts, or by reducing host densities below thresholds necessary to support pathogen populations (Grenfell and Dobson, 1995; Auld and Tinsley, 2015).
Changes to host-pathogen relationships not only will affect specific host-pathogen pairs but also will lead to broader impacts in the surrounding ecosystem. For instance, marine viruses are vital to nutrient cycling and are determiners of planktonic species abundance and distribution (Fuhrman, 1999). If current host-pathogen relationships become distorted or new host-pathogen relationships emerge, community composition and subsequent ecosystem services could be greatly altered. Mortalities of key species may also cause ecological phase shifts due to the loss of three-dimensional structures such as corals or kelp. Ecosystem biodiversity and even species evolution can be influenced by marine pathogens (Suttle, 2007). The effects of climate change on marine host-pathogen dynamics can therefore impact every trophic level of marine ecosystems.
Human health may also be affected by these changing dynamics. Pathogen range expansion and increasing virulence may negatively affect economically important species by impacting fisheries productivity and increasing the risk of human foodborne illness. V. coralliilyticus strains, for example, have shown resistance to commercially available antibiotics (Vizcaino et al., 2010), and range expansion and horizontal gene transfer may allow this trait to become prevalent in other Vibrio species that currently pose no risk to humans. Further investigations of the impact of climate change on Brucella and other marine mammal-borne pathogens are necessary, given the potential for transmission both among species of marine mammals and also to human populations.
Changes in host-pathogen relationships may have negative economic impacts on important commercial fisheries. There will be increased stress on many species as pathogen virulence and host mortality accelerate (Karvonen, 2010). Declining abundance or fitness of target species will result in a decline of subsistence fisheries, and developing nations—particularly those in the tropics—are likely to be among the first impacted (Figure 2).
These examples of changes in host-pathogen relationships show the potential for substantial reductions in biodiversity, loss of valuable ecosystem services, and threats to human health. However, there is a great deal of uncertainty associated with making predictions about the impacts of climate change, and marine host-pathogen systems in particular are understudied and little understood in this regard. In order to meaningfully predict, plan for, and mitigate the potential negative consequences of climate change on these host-pathogen systems, it is necessary that we consider the underlying drivers of change and the likely responses of both pathogens and hosts. Complex interactions among multiple factors, such as rising sea surface temperature, anoxia, ocean acidification, and stratification, require further investigation.