Introduction
Ever since the voyage of HMS Beagle (1831–1836), ocean expeditions have provided novel insights into geological and biological processes. The HMS Challenger expedition (1872–1876) was one of the first to systematically collect numerous marine sediment and organismal samples from around the world (Figure 1), setting the scene for contemporary oceanography, paleoceanography, and marine biology (Macdougall, 2019). The Swedish Albatross expedition (1947–1948) expanded on Challenger insights by extracting the first deep-ocean sediment cores, which encompassed hundreds of thousands of years of sedimentation and facilitated pioneering paleoceanographic and micropaleontological studies (Arrhenius, 1952; Emiliani, 1955; Parker, 1958; Olausson, 1965; Benson and Sylvester-Bradley, 1971; Benson, 1972; Berger, 2011). Sediment cores are vertical columns of sediment recovered by techniques designed to penetrate the seafloor (Figure 1). The Albatross expedition made use of a prototype piston core designed by Kullenberg that was capable of recovering >10 m of sediment (Revelle, 1987).
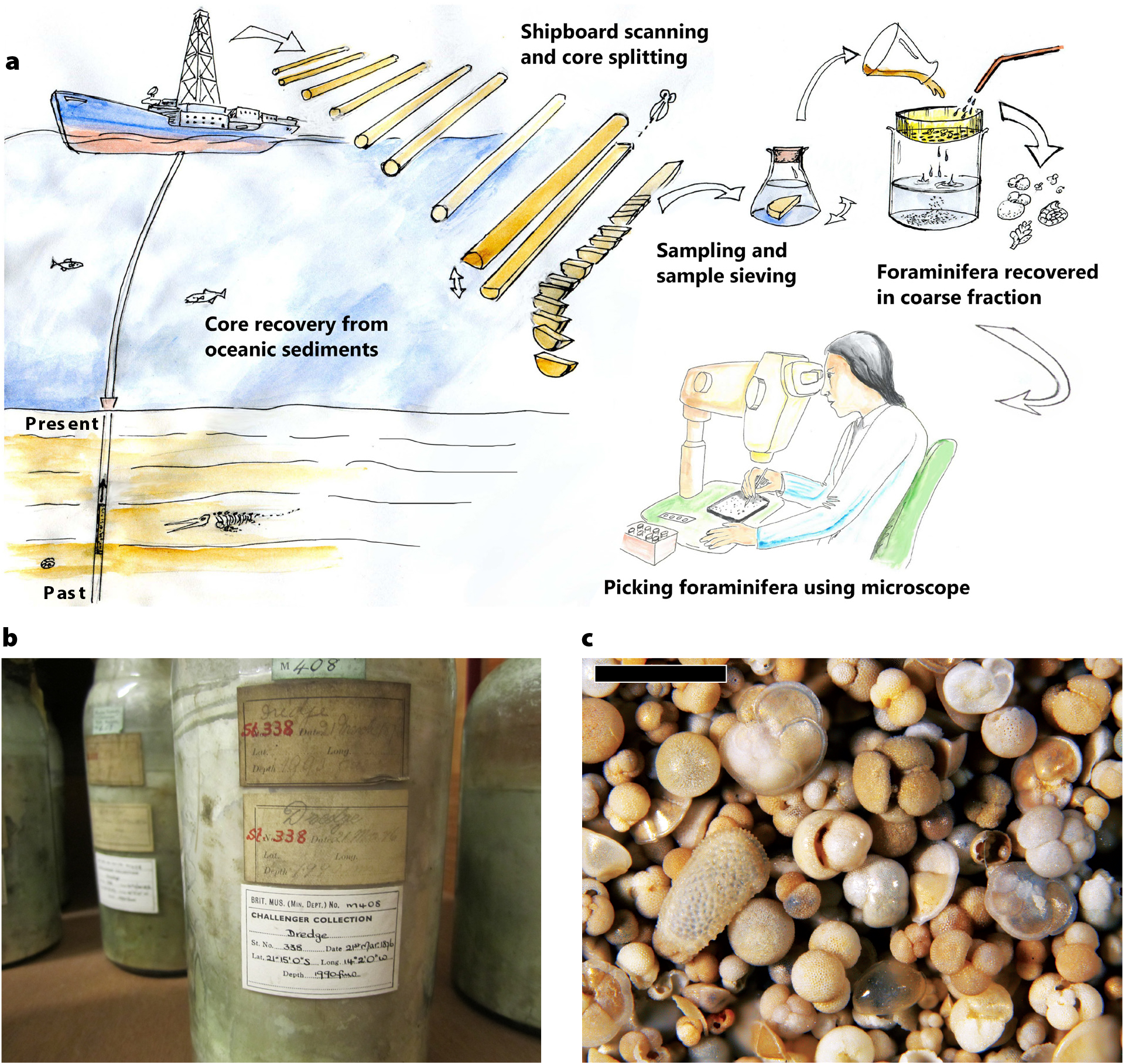
Figure 1. (a) An overview of sediment core collection and processing for micropaleontological research. Illustrations by Simon J. Crowhurst and the Godwin Laboratory. (b) Ocean-floor sediment samples collected with a dredge during the HMS Challenger expedition on March 21, 1876, in the South Atlantic. Sample number M.408 from the Ocean-Bottom Deposit (OBD) Collection held by the Natural History Museum in London (for more information, see Rillo et al., 2019). (c) Sand-sized residue of a Pleistocene deep-sea sediment from ODP Site 925 in the equatorial Atlantic Ocean consisting of numerous microfossil shells. Scale bar: 1 mm. > High res figure
|
Since the Albatross expedition, numerous seafloor sediment samples have been collected from coring expeditions and accumulated at oceanographic institutions (Berger, 2011). These collections have facilitated global-scale analyses of past climate change, such as reconstruction of global ocean conditions during the last ice age by the CLIMAP project (Climate: Long range Investigation, Mapping, and Prediction; CLIMAP Project Members, 1976, 1984). The same material also made it possible to investigate species and community dynamics across temporal scales. For instance, Ruddiman (1969), a geologist, used planktonic foraminiferal records in surface sediments from the North Atlantic to reveal large-scale spatial patterns in present-day species, a pioneering contribution to the field now known as macroecology (Brown and Maurer, 1989; Brown, 1995; Yasuhara et al., 2017b). Ruddiman’s analysis of planktonic foraminiferal diversity was feasible because many paleoclimatic reconstructions, such as CLIMAP, use the present-day distribution and relative abundance of microfossil species as environmental proxies (Box 1). As a result, CLIMAP and related efforts (e.g., the mid-Pliocene PRISM project or Pliocene Research, Interpretation and Synoptic Mapping) have built comprehensive, global data sets of microfossil community censuses for several time periods in Earth history, including the present day, the Last Glacial Maximum, and the Pliocene (CLIMAP Project Members, 1976, 1984; Dowsett et al., 1994, 2013). These data were seldom studied from a biological perspective initially but later proved critical for gaining insight into present (Rutherford et al., 1999; Fenton et al., 2016; Tittensor et al., 2010) and past (Yasuhara et al., 2012c, 2020) biodiversity patterns on global and regional scales.
BOX 1. Microfossils
Microfossils are microscopic remains of organisms or their parts preserved in the fossil record. The most widely studied microfossils are biomineralized structures with high fossilization potential produced by a range of organisms, including photosynthetic plankton such as coccolithophores and diatoms, various mixo- to heterotrophic protists such as planktonic and benthic foraminifera and planktonic radiolarians, and small metazoans such as benthic ostracods (small bivalved crustaceans; Yasuhara et al., 2017b). Dinoflagellates (auto-, mixo-, and heterotrophic plankton) also leave abundant microfossils in the form of resistant organic (and occasionally biomineralized) resting cysts (de Vernal, 2013). Many microfossils represent parts of larger organisms, such as the scales and teeth of fish (Field et al., 2009; Sibert et al., 2017) and shark denticles (Dillon et al., 2017), referred to as ichthyoliths. Although pollen and spores have terrestrial origins, they can also be preserved in both marine and freshwater sediments (Sánchez Goñi et al., 2018).
Microfossils are abundant in deep-sea sediment cores and, in many cases, constitute a large portion of the sediment itself (Figure 1; e.g., see Marsaglia et al., 2015). Thus, hundreds of thousands to millions of plankton specimens can be found in a gram of deep-sea sediment. Microfossils are key tools for interpreting age and environment in sediment cores due to their excellent preservation and high abundance. The first and last appearance of key species and groups are used to determine the ages of sediments in cores (Berggren et al., 1995; Motoyama, 1996), whereas the trace element and isotopic compositions of microfossils are used to reconstruct the paleoceanographic history of sediment cores with regard to, for example, temperature, salinity, and polar ice volume (Figure 2; e.g., Zachos et al., 2001; Lisiecki and Raymo, 2005; Norris et al., 2013). At the same time, the high abundance and diversity of microfossil groups preserved in small amounts of sediment (Figure 1) allow for quantitative assessment of the composition and dynamics of past communities across a range of spatial and temporal scales, as reviewed here.
Although microfossils are ideal subjects for macroecological and macroevolutionary analyses, their study is limited by poor understanding of the life histories and ecology of most species and by evolving species concepts, limited phylogenetic hypotheses for most clades, and the poor preservation potential of other organisms in the community aside from microfossils (i.e., the majority of the ecosystem is not fossilized). In spite of these limitations, almost every trophic or functional group in marine communities is represented by (at least) one well-fossilized microfossil group, providing a basis for macroecological and macroevolutionary synthesis. Notable exceptions are the prokaryotes and viruses, which leave no body fossils. Obtaining information on the history of these groups requires alternative approaches, such as the use of organic biomarkers in the case of some prokaryote clades or ancient DNA (Armbrecht, 2020, in this issue).
|
Scientific ocean drilling began with the launch of the international Deep Sea Drilling Project (DSDP) in 1968, followed by the Ocean Drilling Program (ODP) in 1983, the Integrated Ocean Drilling Program (IODP) in 2003, and the International Ocean Discovery Program (IODP) in 2013 (Becker et al., 2019). These projects allowed scientists to recover sediment sequences up to several kilometers in length, spanning ~170 million years (Figure 1; Becker et al., 2019; Clement and Malone, 2019). Scientific ocean drilling has been deemed one of the most successful international scientific collaborations ever undertaken (Berger, 2011) and has provided unparalleled marine data on a global scale that has resulted in numerous publications (>11,000 peer-reviewed papers; Clement and Malone, 2019; Koppers et al., 2019).
Integration of paleoceanographic and paleobiological data from deep-sea sediments has provided improved understanding of the co-evolution of marine systems and their biota over the last ~10 years. Here, we review these efforts to understand the interaction between climate change and the marine biosphere on both long and short timescales. Our review is time structured and focuses on the influence of climate on biodiversity on million-year, millennial, centennial, and more recent timescales. We end with a discussion of how deep-sea biodiversity dynamics on the most recent timescales can inform our understanding of the changes expected in the Anthropocene.
Biotic Dynamics Over Millions of Years
Quantifying how and why biodiversity levels have changed over Earth history is fundamental to macroecology and macroevolution. Scientific ocean drilling samples have allowed for unprecedented insight into these dynamics on million-year timescales, particularly in response to large-scale global climate and tectonic changes (Figure 2; Kucera and Schönfeld, 2007; Norris, 2000; Fraass et al., 2015; Lowery et al., 2020). The general correspondence between Cenozoic climate change and biodiversity levels across multiple marine clades suggests that climate, particularly temperature, controls diversification dynamics on long timescales (Box 2 and Figure 3). Higher temperatures generally correspond with higher levels of biodiversity (Box 2). However, the precise mechanisms responsible for this coupling are debated. One potential explanation is that higher temperatures can enhance metabolic efficiency and resulting reproduction, with such enhancements potentially resulting in increased speciation and therefore species richness (Allen et al., 2002; Yasuhara and Danovaro, 2016).
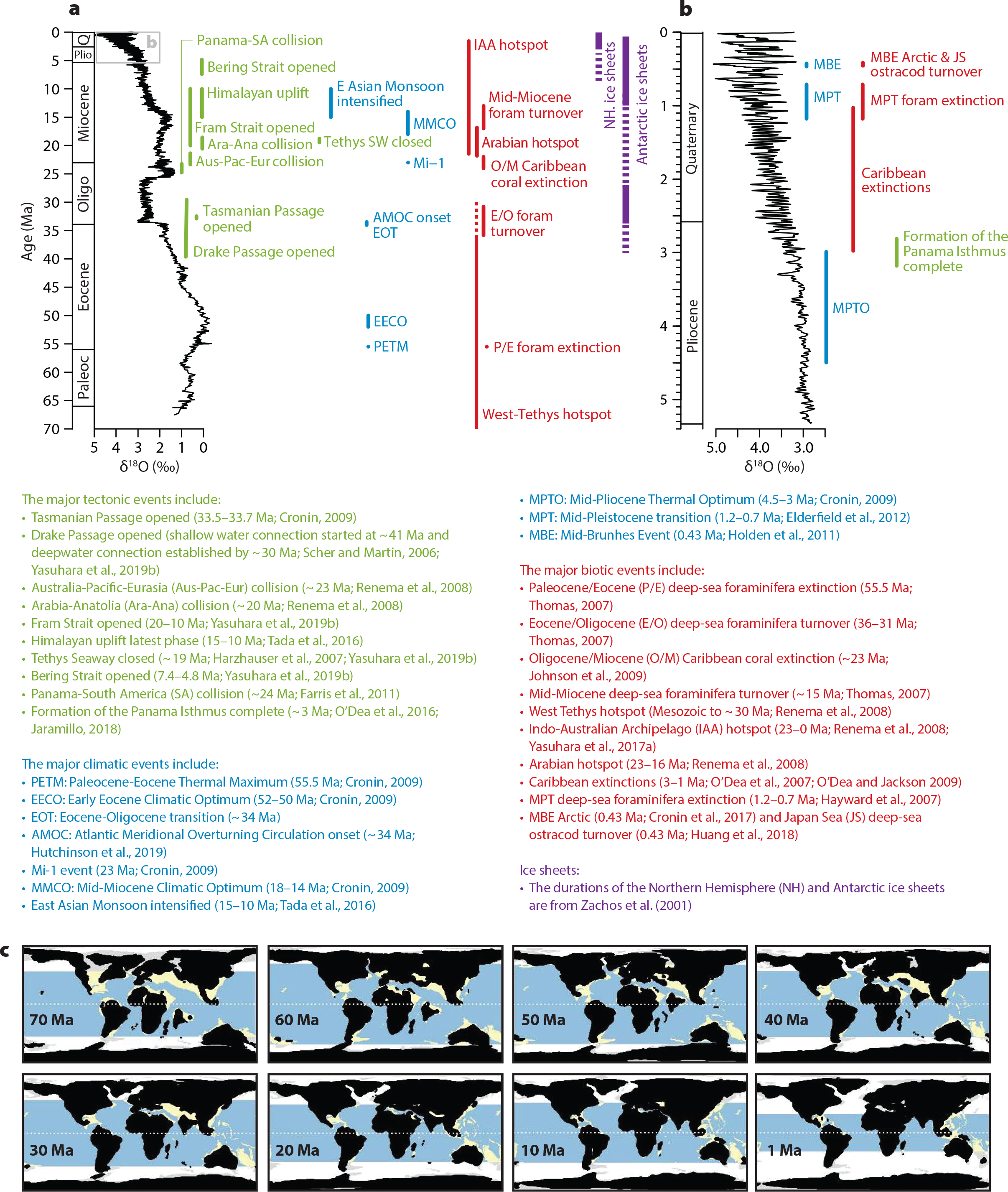
Figure 2. Cenozoic global changes and major events. (a) Cenozoic summary and (b) Plio-Pleistocene closeup. Global deep-sea oxygen isotope records (smaller value indicates warmer climate) are from Zachos et al. (2001) for (a), and from the LR04 stack of Lisiecki and Raymo (2005) for (b). Major tectonic (green), climatic (blue), biotic (red), and ice-sheet (purple) events are shown. (c) Paleogeographic maps from Leprieur et al. (2016). Light blue: deep tropical ocean. Yellow: tropical shallow reefs. White and light gray: deep ocean and shallow waters outside the tropical boundary, respectively. > High res figure
|
BOX 2. Microfossil Biodiversity Trends over the Cenozoic
The Cenozoic biodiversity curve for planktonic foraminifera (Ezard et al., 2011; Fraass et al., 2015) is surprisingly similar to those for other global marine groups, such as sharks (Condamine et al., 2019) and calcareous nannofossils (Lowery et al., 2020; Rabosky and Sorhannus, 2009), and to a regional curve of Neotropical terrestrial plants (Jaramillo et al., 2006) (Figure 3). Commonalities among these curves include: (1) peak biodiversity in the Eocene (56–34 Ma), (2) a major extinction event at the Eocene-Oligocene boundary, (3) a Miocene diversification phase (for planktonic foraminifera and sharks), and (4) a Pliocene biodiversity high (for planktonic foraminifera and sharks) (Figure 3). Dinoflagellates also show a similar Eocene diversity peak (Katz et al., 2005; Stover et al., 1996).
In contrast to calcareous microfossil groups such as planktonic foraminifera and calcareous nannofossils, siliceous microfossil groups such as diatoms and radiolarians show contrasting biodiversity trends (Lowery et al., 2020). For example, diatom diversity increased during cooling periods, peaked at the Eocene-Oligocene transition, and reached highest levels during the Plio-Pleistocene (Figure 3; Katz et al., 2005, 2007; Lazarus et al., 2014; Lowery et al., 2020). Cetaceans show trends similar to those of diatoms, and the two may have coevolved (Berger, 2007; Marx and Uhen, 2010). The contrast between calcareous and siliceous microfossil biodiversity patterns may occur because calcareous microfossils tend to be dominant and diverse in tropical and subtropical latitudes, whereas siliceous groups are dominant in polar, high latitude seas (Rutherford et al., 1999; Powell and Glazier, 2017; Dutkiewicz et al., 2020; Lowery et al., 2020). Thus, colder periods may allow for higher biodiversity in siliceous microfossil groups, given their preference for cooler waters. Alternatively, the differences could also reflect biases resulting from preservation and sampling of siliceous microfossils (Lowery et al., 2020).
|
In addition to regulating temporal patterns of biodiversity, climate change over the Cenozoic has affected spatial patterns of marine biodiversity. For example, large-scale climatic shifts have modified one of the foremost patterns in ecology, the latitudinal biodiversity gradient (LBG), in which the number of species decreases from the equator to the poles (Hillebrand, 2004a,b; Saupe et al., 2019). In the ocean, LBGs are often characterized by an equatorial dip, resulting in a bimodal biodiversity pattern (Rutherford et al., 1999; Worm et al., 2005; Chaudhary et al., 2016, 2017; Worm and Tittensor, 2018; Rogers et al., 2020; Yasuhara et al., 2020). Growing evidence suggests the LBG was flatter during warm periods (e.g., Eocene, Pliocene) and steeper during cold periods (e.g., Last Glacial Maximum of 20,000 years ago; Yasuhara et al., 2012c; Fenton et al., 2016; Lam and Leckie, 2020; Meseguer and Condamine, 2020), potentially reflecting degree of climatic heterogeneity (Saupe et al., 2019). The standard tropical-high and extratropical-low LBG can be traced back at least to the Eocene for both deep-sea benthos (~37 million years ago [Ma]; Thomas and Gooday, 1996; Figure 3) and pelagic plankton (~48–34 Ma; Fenton et al., 2016). Notably, initiation of the LBG observed today in the deep sea predates the Eocene-Oligocene transition (Thomas and Gooday, 1996), suggesting that it began with the opening of the Drake Passage (Scher and Martin, 2006; Figure 2) rather than with climatic changes at the Eocene-Oligocene transition (Figure 3). Indeed, the opening and closing of major seaways (Figure 2) has been shown to alter the distribution of both shallow-marine and deep-sea organisms throughout the Cenozoic, both longitudinally (e.g., Tethyan and Central American Seaways) and latitudinally (e.g., Arctic gateways) (O’Dea et al., 2007; Renema et al., 2008; Yasuhara et al., 2019b).
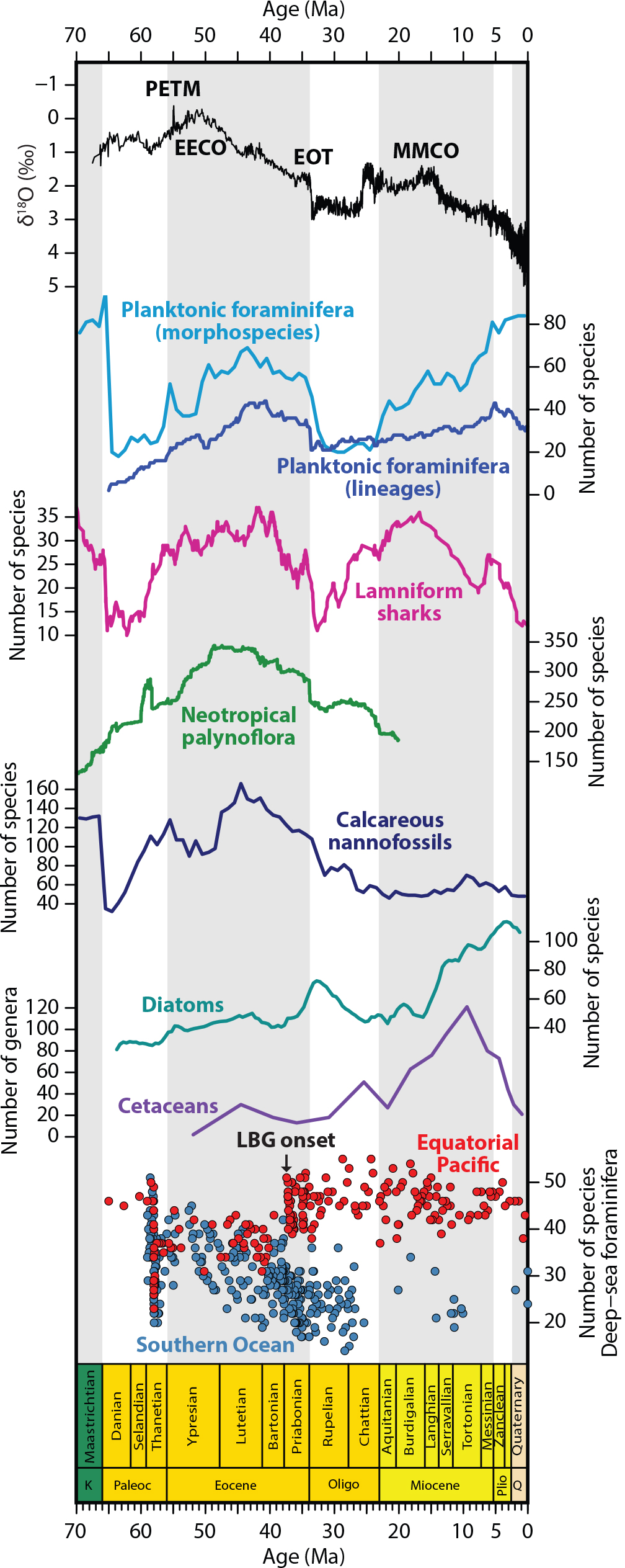
Figure 3. Cenozoic climate and biodiversity estimates of multiple taxonomic groups. From top to bottom: oxygen isotopic records (Zachos et al., 2001; smaller value indicates warmer climate) and biodiversity curves of global planktonic foraminifera morphospecies (Lowery et al., 2020) and lineages (Ezard et al., 2011), global lamniform sharks (Condamine et al., 2019), neotropic palynoflora (Jaramillo et al., 2006), global calcareous nannofossils (Lowery et al., 2020), global diatoms (Lazarus et al., 2014), global cetacean genera (Marx and Uhen, 2010; Uhen, 2020), and Southern Ocean and equatorial Pacific deep-sea benthic foraminifera (Thomas and Gooday, 1996). The onset of the deep-sea benthic foraminifera latitudinal biodiversity gradient (LBG) is indicated at ~37 million years ago. > High res figure
|
Although evidence supports climate as a primary control on Cenozoic biodiversity change, it is certainly not the only driver of biodiversity dynamics (e.g., Ezard et al., 2011; Condamine et al., 2019; Lam and Leckie, 2020). Ecological interactions in addition to climate, for example, have been found to influence the macroevolution of planktonic foraminifera (Ezard et al., 2011). Continued study of biotic traits will allow for examination of the relative roles of abiotic (e.g., climate) versus biotic factors in shaping ecosystems and their changes through time (Schmidt et al., 2004), as well as of the relationship between biodiversity and ecosystem function (Henehan et al., 2016; Yasuhara et al., 2016; Alvarez et al., 2019).
Biotic Dynamics on Millennial Timescales
In addition to million-year timescales, sediment core data provide insight into biodiversity-climate dynamics on millennial timescales. Orbital variations have resulted in changes in climate on 10,000- to 100,000-year timescales throughout Earth history, as is well documented in benthic δ18O records (Figure 2B; Raymo et al., 2004; Lisiecki and Raymo, 2005). Surface-ocean and deep-ocean conditions change in pace with orbital forcing of global climate, as do the locations of oceanic currents and bio-provinces (Cronin, 2009). These oceanic changes are matched by changes in the composition of marine microfossil communities that mirror orbital-scale climatic changes (Cronin et al., 1996, 1999; Cronin and Raymo, 1997). Orbital forcing provides repeated experiments on community assembly, with microfossil assemblages demonstrating that similar communities come together repeatedly under similar environmental conditions (Cronin et al., 1996; Beaufort et al., 1997; Yasuhara and Cronin, 2008; Huang et al., 2018). This close association supports climate, and particularly temperature, as a key driver of marine biodiversity, with the formation of similar assemblages reflecting the process of species tracking their preferred temperature conditions. Orbital-scale time-series studies, for example, consistently show that temperature rather than productivity is the best predictor of deep-sea biodiversity patterns (Hunt et al., 2005; Yasuhara et al., 2009, 2012b). Temperature likely controls biodiversity because fewer species can physiologically tolerate conditions in colder places than in warmer places on these ecological timescales (Currie et al., 2004; Yasuhara and Danovaro, 2016).
Changes in the dominant mode of orbital cyclicity can, by contrast, permanently disturb marine ecosystems (Hayward et al., 2007; DeNinno et al., 2015; Cronin et al., 2017; Huang et al., 2018, 2019), such as during the transition from 41,000- to 100,000-year cycles in the Mid-Pleistocene Transition (MPT) and the Mid-Brunhes Event (MBE). For example, deep-sea benthic foraminifera show a prominent global extinction event during the MPT (Hayward et al., 2007). Similarly, ostracod taxa with affinity for warm temperatures were abundant both in the Arctic and the North Atlantic Oceans before the MBE (DeNinno et al., 2015; Cronin et al., 2017). However, after the MBE warm-adapted taxa went extinct regionally in the Arctic, with shrinking distributions to the south (DeNinno et al., 2015; Cronin et al., 2017). In the Sea of Japan, endemic cool water species replaced circumpolar species after the MBE, and many circumpolar species went extinct regionally (Ozawa and Kamiya, 2005; Cronin and Ikeya, 1987; Huang et al., 2018, 2019). In sum, when orbital cyclicity is consistent, microfossil species seem to show evidence of repeated community assembly that matches prevailing conditions, indicating that community assembly may be deterministic. Changes in the expression of orbital forcing, however, can lead to extinction. How and why such changes instigate widespread biotic disturbance is not well understood, but may be explained by the scale of environmental perturbation; larger-scale changes could exceed species’ tolerances and/or eliminate potential refugia (Hayward et al., 2012).
Biotic Dynamics on Centennial Timescales
Recent advances in high-resolution paleoceanographic studies (Bond et al., 1997; Bianchi and McCave, 1999; deMenocal et al., 2000; Oppo et al., 2003; McManus et al., 2004; Yasuhara et al., 2019a) have improved understanding of centennial-scale biotic responses to climate change, bridging the gap between geological timescales and the timescales of ecological studies. The centennial timescale has long been a “blind spot” in ecological analysis, lodged between the range of biological monitoring without historical reconstruction and the resolution attainable in most paleontological research (Yasuhara, 2019). Paleobiological records on this timescale can be garnered from microfossils preserved in sediments deposited under high sedimentation rates and/or conditions that minimize post-depositional sediment mixing. Such records can be found in sediment drifts (e.g., sediments collected from a sediment drift at ODP Site 1055 on the Carolina Slope show sedimentation rates of ~23 cm per thousand years; Yasuhara et al., 2008) or in enclosed settings in marginal oceanic basins that act as natural sediment traps and where local anoxia prevents sediment mixing (such as the basins of the Californian borderland, Cariaco Basin, or silled fjords; see Yasuhara et al., 2019c). Although seasonal and annual signals are likely smoothed by bioturbation in many of these sediment cores, it is minimized in cores from anoxic basins. By using these minimally disturbed cores, decadal community changes can be reconstructed to bridge the gaps across timescales (Kuwae et al., 2017; Salvatteci et al., 2018; see the next section).
Pioneering studies using records from the Gulf of Mexico and the Santa Barbara Basin off California have documented responses of biodiversity to centennial-scale abrupt climatic changes (Flower and Kennett, 1995; Cannariato et al., 1999). Initial findings documenting the response of benthic foraminifera to centennial-scale deoxygenation events in the Santa Barbara Basin (Cannariato et al., 1999) have been complemented by data from various benthic groups, including molluscs, foraminifera, ostracods, and ophiuroids (Moffitt et al., 2015; Myhre et al., 2017). Centennial-scale records spanning the last 20,000 years in the North Atlantic Ocean have revealed that deep-sea benthic ostracod diversity responded to changes in deepwater circulation and temperature during the abrupt climatic changes of the Heinrich I (17,000–14,600 yr BP), the Younger Dryas (12,900–11,700 yr BP), and the 8.2 ka event (8200 yr BP) without recognizable time lags (Yasuhara et al., 2008, 2014; Yasuhara, 2019). These studies also documented rapid rearrangement of local communities following the abrupt climatic changes. Thus, even on centennial timescales, climate, and more specifically temperature, has dramatic effects on marine biodiversity. At least locally, the dominant response to climate change seems to involve range shifts and recolonization from the same species pool (Yasuhara and Cronin, 2008; Yasuhara et al., 2009; Yasuhara and Danovaro, 2016). Excessive extinctions in the future may therefore affect the resilience of these ecosystems.
BOX 3. Automation
Another advantage of the microfossil record is that it is increasingly possible to automate key steps in gathering and processing data, due in part to the small size of samples and specimens. Once a core is obtained, the major data-gathering steps are washing and sieving sediment; picking, identifying, and mounting specimens; and, for studies of phenotypic evolution, measuring morphological traits of specimens. Recent technological and methodological advances can substantially reduce the time and effort required for some of these steps.
Automated picking systems that take sieved size fractions, separate them into individual particles, and image each particle may greatly reduce picking times (de Garidel-Thoron et al., 2017; Itaki et al., 2020). With samples that have already been picked and mounted, hundreds or thousands of individual microfossils can be imaged simultaneously in three dimensions and algorithmically parsed into individual images from which basic morphometric traits and features can be automatically extracted and analyzed at the assemblage scale (Beaufort et al., 2014; Elder et al., 2018; Hsiang et al., 2018, 2019; Kahanamoku et al., 2018).
These efforts build on decades of previous automation work that either extracted coarser (size related) data or was relatively more labor intensive (Bollmann et al., 2005; Knappertsbusch et al., 2009). Given sufficient training data sets, convolutional neural nets can now identify planktonic foraminifera, coccolithophores, and radiolarians with accuracy similar to that of taxonomic specialists (Beaufort and Dollfus, 2004; de Garidel-Thoron et al., 2017; Hsiang et al., 2019; Itaki et al., 2020). Given these ongoing developments, it is becoming possible to envision a near future in which the entire sample processing and data extraction workflow is streamlined and largely automated, with taxonomic experts guiding and overseeing the process but spending the majority of their time analyzing data sets that may be far larger, denser, and more data-rich than is currently feasible.
|
Microfossils and the Brave New Anthropocene
The sediment layer on the surface of the ocean floor represents a time-averaged assemblage of microfossils. Because of slow sedimentation in the deep sea (typically less than 10 cm per 1,000 years) and sediment mixing down to a depth of 10 cm (bioturbation), a typical 1 cm thick surface-sediment sample represents average deposition over centuries to millennia (Jonkers et al., 2019). Thus, the proportion of microfossils recording conditions of the Anthropocene (typically >~1950) in surface sediments is negligible, which means core-top sediments typically provide a global pre-industrial baseline for the state of marine communities in fossilized organisms (Jonkers et al., 2019; Yasuhara et al., 2020).
Recently, Jonkers et al. (2019) compared planktonic foraminifera assemblages collected from surface sediments that provide a pre-industrial baseline with assemblages collected from sediment traps that monitored particle flux to the seafloor over the last 40 years. The authors examined whether anthropogenic climate change modified the composition of marine plankton communities. They found that Anthropocene assemblages differ from their pre-industrial equivalents, and the observed differences in species composition are consistent with the expected effect of current temperature change trends (Jonkers et al. 2019). Similarly, Moy et al. (2009) used shells of planktonic foraminifera from surface sediments as a benchmark for calcification intensity in living planktonic foraminifera. These authors discovered that shells of modern Globigerina bulloides are about a third lighter than those from the sediments, consistent with reduced calcification induced by ocean acidification in the Anthropocene. Their results were recently confirmed by Fox et al. (2020), who observed shell thinning when comparing planktonic foraminifera specimens collected from historical (HMS Challenger, 1872–1876) to recent (Tara Oceans, 2009–2016) plankton samples. Large collections of surface sediment samples, both modern and historical (Rillo et al., 2019), are available to study, providing an opportunity to quantify anthropogenic impacts on the composition of a range of marine ecosystems and on traits of their constituent species.
Areas with particularly high rates of sedimentation (e.g., ~50–100 cm per hundred years in Osaka Bay and >120 cm per thousand years in the Santa Barbara Basin; Barron et al., 2010; Field et al., 2006; Yasuhara et al., 2007) can provide insight into the effects that humans are having on marine ecosystems on even finer temporal scales. Study of these sediments has revealed significant marginal marine ecosystem degradation caused by human-induced eutrophication and resulting bottom water hypoxia (Barmawidjaja et al., 1995; Cooper, 1995; Cronin and Vann, 2003; Weckström et al., 2007; Willard and Cronin, 2007; Yasuhara et al., 2007, 2019c; Tsujimoto et al., 2008).
Although anthropogenic forcing is the primary driver of current biodiversity change (Díaz et al., 2019), natural variability in community composition is also at play, and its overprinting can prevent quantification of anthropogenic effects. Measuring this baseline temporal variability is crucial to partition the human signal, but is difficult to accomplish without long ecological time series that precede the Anthropocene. Microfossils provide these time-series data on community composition across multiple temporal scales, albeit for a limited set of taxa. For example, using planktonic foraminifera data, Lewandowska et al. (2020) compared the magnitude of biodiversity change across temporal scales from decades to millions of years. They found that, as expected, biodiversity change was greatest across the longest multi-million-year timescale and decreased at shorter timescales. However, they observed relatively large changes in community composition, comparable to the magnitude of changes over the longest timescale, most recently. The magnitude of recent turnover is suggestive of a large anthropogenic effect but may also reflect “noisy” annually averaged sediment trap time series.
Fish-scale paleobiological studies have similarly provided insight on baseline variability in fish populations. For example, marginal marine and continental margin sediments in the Pacific extended population dynamics for anchovy and sardines back to the nineteenth century and past millennia (Baumgartner et al., 1992; Field et al., 2009; Checkley et al., 2017; Kuwae et al., 2017; Salvatteci et al., 2018). These records show that fish population dynamics are more complex and region-specific than those perceived based on twentieth-century fishery data, with a clear Pacific-wide correlation between anchovy and sardine populations and Pacific Decadal Oscillations (Chavez et al., 2003; Kuwae et al., 2017; Salvatteci et al., 2018). In sum, modern and historical time series of long-term biological monitoring are limited (e.g., Chavez et al., 2003; Engelhard, 2005; Lotze and McClenachan, 2014), especially in the tropics (Dornelas et al., 2018; Blowes et al., 2019), but those that are available are extremely valuable for comparing the magnitude of biodiversity change observed in the fossil record to that observed in response to anthropogenic and recent climatic forcing.
“While we lack a true time machine, the opportunity provided by this
“biological time machine” remains unique.”
|
Future Outlook
The continuity and duration of marine sediment core data make it possible to assess the relative importance of abrupt versus gradual, secular changes in climate to species and communities, tied to a refined (and ever improving) understanding of past climate change. The importance of spatial and temporal scales in (macro)ecology and (macro)evolution is well known (Brown and Maurer, 1989; Benton, 2009; Blois et al., 2013), with the patterns and drivers differing across space (Chiu et al., 2019; Jöst et al., 2019; Kusumoto et al., 2020) and time (Huang et al., 2018; Yasuhara et al., 2016, 2019b). Marine sediment cores permit interrogation of these dynamics at multiple temporal scales (Lewandowska et al., 2020). Biotic interactions generally tend to control dynamics on smaller spatial and temporal scales, while physical climatic factors, particularly temperature as reviewed here, appear to dominate biotic dynamics on larger scales (Benton, 2009; Yasuhara et al., 2016). However, there are gaps in our theoretical understanding of why this occurs, of the temporal scales on which physical factors begin to dominate, and of the relative importance of short abrupt events, cyclical changes, and long-term secular trends in shaping species and ecosystems. Continued study of marine sediment cores can help to fill these gaps, particularly with concerted comparisons among Anthropocene, centennial, millennial, and million-year timescales.
Regardless of timescale, paleobiological studies, especially those that examine the relationship between climate and biodiversity and ecosystem functioning, provide insight into the potential response of biodiversity to ongoing climate change. The pace and scale of anthropogenic impacts on ecosystems and ecosystem services remain of great concern (Díaz et al., 2019). Climate change is expected to have an accelerating effect on the ocean, yet the challenges of using relatively short-term ecological data to understand long-term consequences to biodiversity and ecosystems remain significant. Sediment cores and associated microfossils can help elucidate links between climate and spatial biodiversity (e.g., Yasuhara et al., 2012c, 2020), extinction risks (e.g., Harnik et al., 2012; Finnegan et al., 2015), natural baselines (e.g., Yasuhara et al., 2012a, 2017b), and biotic consequences on evolutionary timescales (e.g., Ezard et al., 2011).
Yet, linking paleoecological insights to modern-day ecological change is relatively unexplored, and such insights do not necessarily make their way into informing global policy. Identifying and circumscribing the limits of such transposition remain challenging, particularly given the taxonomic biases of preservation and the rapidity of modern-day change. The opportunity remains to address such challenges and ensure that paleoecological data complement modern ecological data and, where appropriate, contribute to assessments and policy (e.g., IPBES [Intergovernmental Science-Policy Platform on Biodiversity and Ecosystem Services], the post-2020 Global Biodiversity Framework of the CBD [Convention on Biological Diversity]). Studies of sediment cores provide a long-term perspective on climate/biodiversity links that can contextualize modern marine ecological change and provide insights that would otherwise remain absent.
Acknowledgments
We thank Katsunori Kimoto, Jeremy R. Young, Kotaro Hirose, Tamotsu Nagumo, Yoshiaki Aita, Noritoshi Suzuki, David Lazarus, André Rochon, Erin M. Dillon, and Briony Mamo for help with microfossil images; Simon J. Crowhurst and David A. Hodell for help with coring overview illustrations; Richard D. Norris, R. Mark Leckie, and Peggy Delaney for thoughtful comments; and guest editors Peggy Delaney, Alan C. Mix, Laurie Menviel, Katrin J. Meissner, and Amelia E. Shevenell for editing and for the invitation to contribute to this special issue. This work is a product of the PSEEDS (Paleobiology as the Synthetic Ecological, Evolutionary, and Diversity Sciences) project, and is partly supported by grants from the Research Grants Council of the Ho‑Kong Special Administrative Region, China (Project No. HKU 17302518, HKU 17311316, HKU 17303115), the Seed Funding Programme for Basic Research of the University of Hong Kong (project codes: 201811159076, 201711159057), and the Faculty of Science RAE Improvement Fund of the University of Hong Kong (to M.Y.).