Introduction
Eastern boundary upwelling systems represent one of the ocean’s most productive biomes, supporting some one-fifth of the world’s wild marine fish harvests (Pauly and Christensen, 1995). As one of the ocean’s four major eastern boundary upwelling systems, the California Current Large Marine Ecosystem (CCLME) is highly valued for the suite of ecosystem services that it provides, and it is the subject of extensive management and policy-focused monitoring and research efforts (see Lubchenco et al., 2019, in this issue). In contrast to open-ocean ecosystems, coastal ecosystems such as the CCLME are highly dynamic physically, biogeochemically, and ecologically. Previously, this dynamism was generally thought to obscure the near-term expression of global change impacts (Hales et al., 2005; Frölicher et al., 2016). But today, coastal upwelling ecosystems are viewed as early indicators of global change impacts (Doney et al., 2011). In fact, the US west coast now sits at the forefront of science and science-to-policy engagement for understanding and addressing the challenges of ocean acidification and hypoxia. What steps led to these changes? Are there lessons that can be drawn to enhance understanding of global change impacts in other systems? And what research, monitoring, and policy actions will enable continued progress as these changes continue to unfold?
Although it is now widely recognized that ocean acidification (OA) and hypoxia (H) are linked in the CCLME (Feely et al., 2016), the progression of the study of these global processes followed different tracks. Hypoxia (dissolved oxygen ≤1.4 ml l–1; Box 1) emerged as a local issue in the northern portion of the CCLME, and our understanding of the issue gradually increased in scale through time toward our current understanding of global deoxygenation and the coupled nature of OAH in upwelling systems. In contrast, OA was widely understood as a global issue prior to its recognition as a particularly serious local threat in the CCLME. As scientists moved from a global understanding of OA to a regional and then more local focus on the CCLME, questions regarding the drivers and consequences of spatial and temporal variability in environment have expanded.
Sudden Appearance of Upwelling-Driven Hypoxia
Hypoxia has long been recognized as a problem for estuaries, semi-enclosed seas, and shelf regions near major river outfalls where land-based nutrient inputs exert their greatest influence on eutrophication (Diaz and Rosenberg, 2008). In the open ocean, oxygen minimum zones (OMZs) were well documented globally and found to be broadly distributed in the eastern Pacific (Helly and Levin, 2004). The dissolved oxygen (DO) concentrations in these OMZ regions reach near zero and are found well below 500 m (Helly and Levin, 2004), thus fairly distant from the nearshore, shallow waters of the continental shelf. A defining physical feature of the CCLME is coastal upwelling, which brings water from above the OMZ (~200 m depth) to the continental shelf. These waters are moderately oxygen-poor, though not typically hypoxic, and are nutrient-rich. For the CCLME, the absence of nearshore, inner-shelf (water depths less than 50 m) hypoxia changed in 2002 with reports of crab mortality from the Dungeness crab fishery (Box 2), the most valuable fishery in the region, yielding, for example, over $50 million annually in landings off Oregon alone. At that time, there was no continuous in situ DO monitoring taking place in the northern CCLME. However, ship-based observations made by the Partnership for Interdisciplinary Studies of Coastal Oceans (PISCO), the Global Ocean Ecosystem Dynamics (GLOBEC) program, and the Oregon Department of Fish and Wildlife (ODFW) revealed a hypoxic zone that extended along 50 km of the coast and stretched landward across the continental shelf into the nearshore (Grantham et al., 2004). Initially, this was thought to be a local-scale phenomenon, driven by the confluence of a strong local phytoplankton bloom that heightened respiratory oxygen demands near the seafloor and quiescent winds that slowed air-sea oxygen exchange. This view changed as observations in the North Pacific pointed to an anomalous intrusion of nutrient-rich subarctic waters that enhanced phytoplankton blooms (Freeland et al., 2003). As phytoplankton cells sank, they were fed upon by consumers such as microbes that heightened the respiratory demands for DO in ocean waters below the surface across the Northeast Pacific. Because water upwelled to the Oregon shelf was already anomalously depleted in DO (Grantham et al., 2004), and weak and retentive currents over sectors of the Oregon shelf held this hypoxic water close to shore (Barth et al., 2005), DO decreased below biologically critical thresholds and resulted in extensive die-off of marine life.
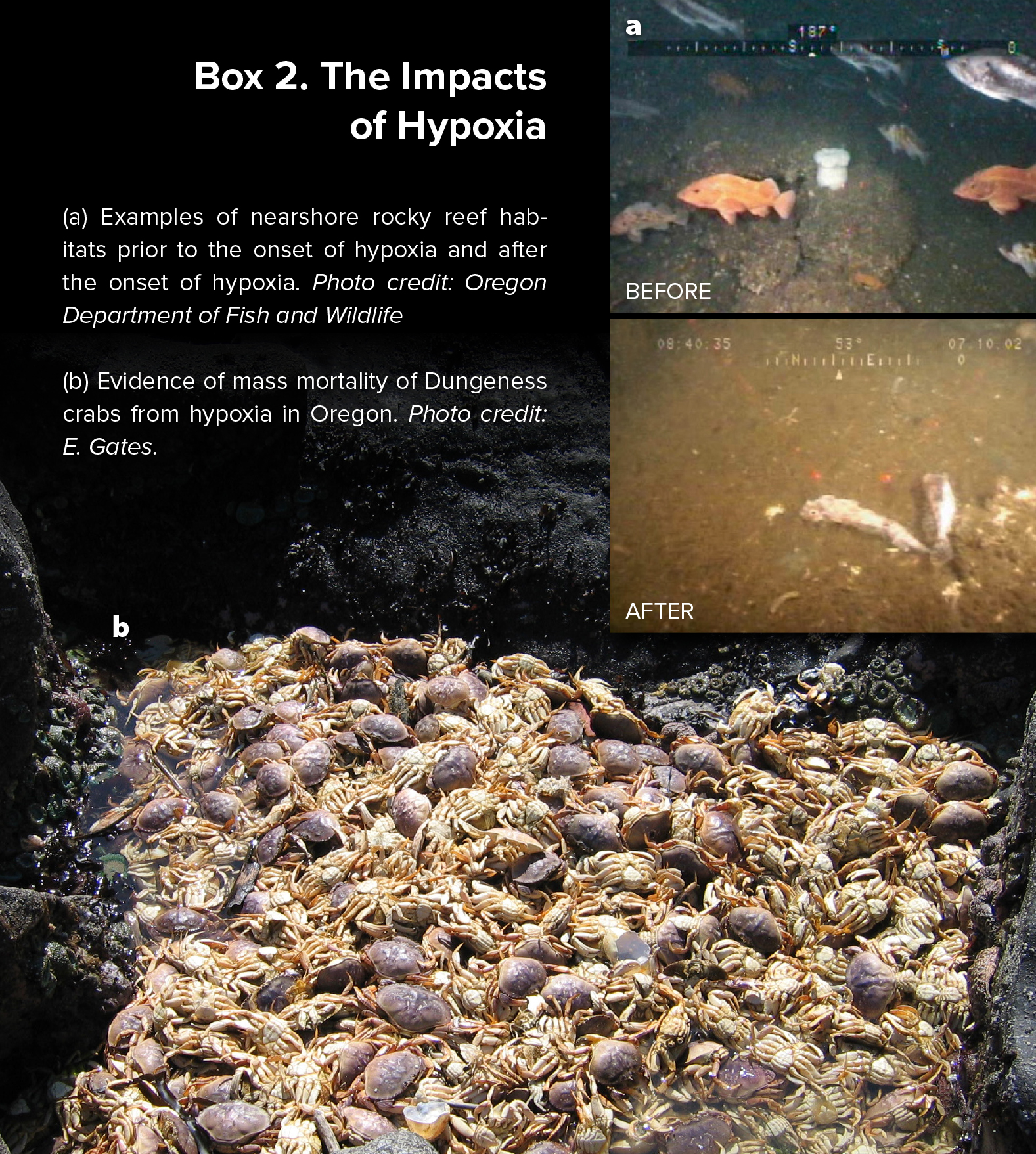
|
The coastal waters of the northern CCLME represent an extremely challenging environment for sustained deployment of oceanographic instruments. The shallow inner-shelf depths require the use of small but less stable vessels, and exceptional rates of biological productivity translate into enhanced rates of instrument biofouling. In 2003, PISCO initiated ship- and mooring-based time-series observations to better capture the potential for, or reality of, shelf hypoxia. The need for continuous observations was evident as hypoxia was revealed to be a highly dynamic, event-scale phenomenon in the inner shelf, where DO concentrations can range from super-saturation to suboxia at semidiurnal to weekly timescales (Figure 1). This temporal variation also exhibits a strong cross-shelf gradient, where the event-scale dynamism of the inner shelf gives way to season-scale persistence of hypoxia in the mid-shelf (Figure 1). The contrast in DO dynamics between the shelf and the shelf break further highlights the important role that local biogeochemical and physical processes play in structuring ecosystem sensitivity to hypoxia. Recognition of the spatial and temporal nature of exposure to low DO water continues to shape our understanding of biological vulnerability to coastal hypoxia.
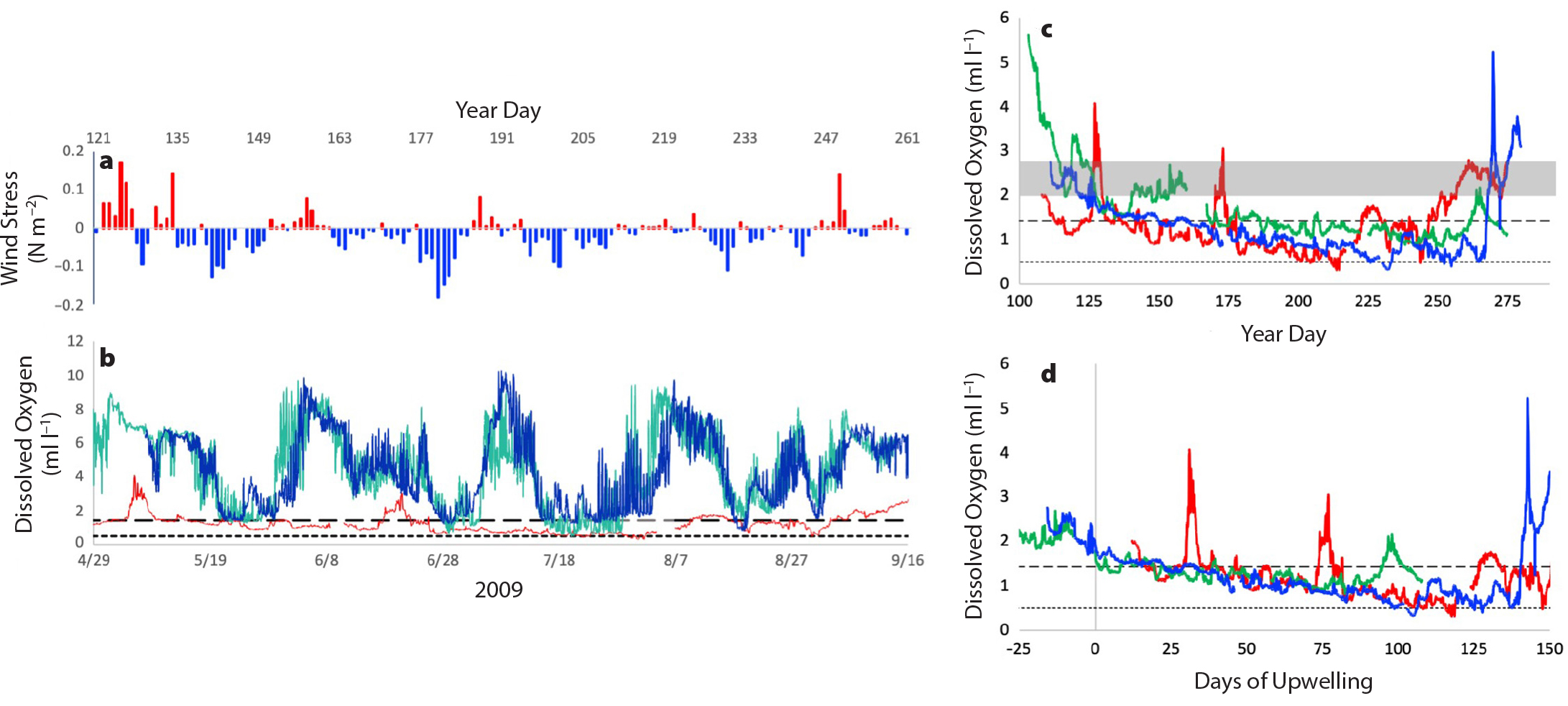
Figure 1. Examples of (a) intraseasonal variation in upwelling wind stress (44.65°N) and (b) near-bottom dissolved oxygen (DO) from Partnership for Interdisciplinary Studies of Coastal Oceans (PISCO) moorings at 44.25°N at a mid-shelf (70 m depth) station (red) and at inner-shelf (15 m) stations at 44.25°N (teal) and 44.86°N (blue), illustrating cross-shelf and alongshore differences in the variability and intensity of hypoxia exposure. Dashed and dotted lines denote 1.4 ml l–1 hypoxia and 0.5 ml l–1 severe hypoxia thresholds, respectively. (c) Interannual variations in near-bottom density and DO from the 70 m mid-shelf station (44.25°N). The shaded region marks the DO concentration of upwelled water at the shelf break, illustrating the importance of shelf respiration in driving the system to hypoxia. (d) The same DO time series as (c), but aligned with the onset of the spring transition, illustrating ways sustained observations can improve the understanding of DO dynamics. Figures modified from Adams et al. (2013). > High res figure
|
The 2002 event helped to catalyze what was to become a rapidly improving understanding of the linkages between broad-scale, climate-dependent changes in ocean chemistry and circulation and oxygen dynamics in coastal upwelling systems. Severe hypoxia, defined as DO ≤0.5 ml l–1, became a recurring feature in shelf waters despite the absence of such low values in the previous five decades of observations in the system. The most severe event occurred in 2006, when exceptionally intense upwelling of anomalously low DO waters drove the novel emergence of shelf anoxia (Chan et al., 2008). Importantly, these events took place within the context of new research from offshore and open ocean time-series observations that began to reveal broad-scale trends of DO declines (Whitney et al., 2007; Stramma et al., 2008). Termed “ocean deoxygenation,” low DO is now understood to be a highly dynamic variable rather than a relatively static property of the global ocean. New research indicated that oxygen concentrations in the ocean are responsive to anthropogenic climate change through the direct effects of warming on gas solubility, and through the indirect effects of warming on the supply of oxygen to deep ocean layers and of ocean circulation (Breitburg et al., 2018). In upwelling systems, such DO declines in oceanic source waters translate into an amplified risk for hypoxia. As water transits across productive shelf waters, respiration by microbes further draws down DO, leading to hypoxia and even anoxia.
Simultaneous with efforts to collect time series of dissolved oxygen at selected subsurface locations off the Oregon coast, including those by PISCO, other groups were collecting and analyzing subsurface oxygen data from large-scale spatial surveys off Oregon and elsewhere in the CCLME (e.g., work by NOAA’s Northwest Fisheries Science Center off Oregon and Washington and by Canada’s Department of Fisheries and Oceans further north off Vancouver Island). These surveys included cross-shelf and vertical sections of DO measured by an underwater ocean glider off Newport, Oregon, that showed the two-dimensional extent of the hypoxia zone off central Oregon (Adams et al., 2013). Using data from a variety of NOAA surveys, Peterson et al. (2013) identified regions of hypoxia that tended to occur north of 42°N and that were most severe over the widest areas of the continental shelf. Hypoxic waters covered up to 62% (15,600 km2) of the continental shelf during some years and <10% in others.
As summarized above, anthropogenic effects on upwelling are important drivers of severe hypoxia at the local shelf ecosystem scale. It is well known that wind-driven upwelling draws low DO, but nutrient-rich, water onto the continental shelf, and influences the time of water retention in the inner shelf. As first proposed by Bakun (1990), climate change is hypothesized to enhance land-sea atmospheric pressure differences that translate into increases in upwelling-favorable wind stress. This process increases nutrient supply and enhances local respiratory DO loss, thereby increasing the risk of hypoxia and anoxia in upwelling systems (Bakun et al., 2010). The emergence of anoxia in 2006 in response to a doubling of upwelling wind stress supports a key prediction of this scenario (Chan et al., 2008). In addition, although seasonal onset of hypoxia can vary greatly from year to year, through sustained observations, a picture of a strong and surprisingly predictable linkage between the start of upwelling winds and the emergence of shelf hypoxia later in the upwelling season has become apparent (Figure 1). Interestingly, signals of long-term increase in upwelling wind stress have yet to emerge for all parts of the CCLME. A possible explanation is Rykaczewski et al.’s (2015) amendment to Bakun’s original hypothesis suggesting that poleward movement of oceanic high-pressure cells may be central in modulating climate-driven increases not just for upwelling but also for its spatial variability. In this way, the risk of climate-driven intensification of hypoxia can be viewed as the product of ocean deoxygenation and upwelling intensification (Figure 2).
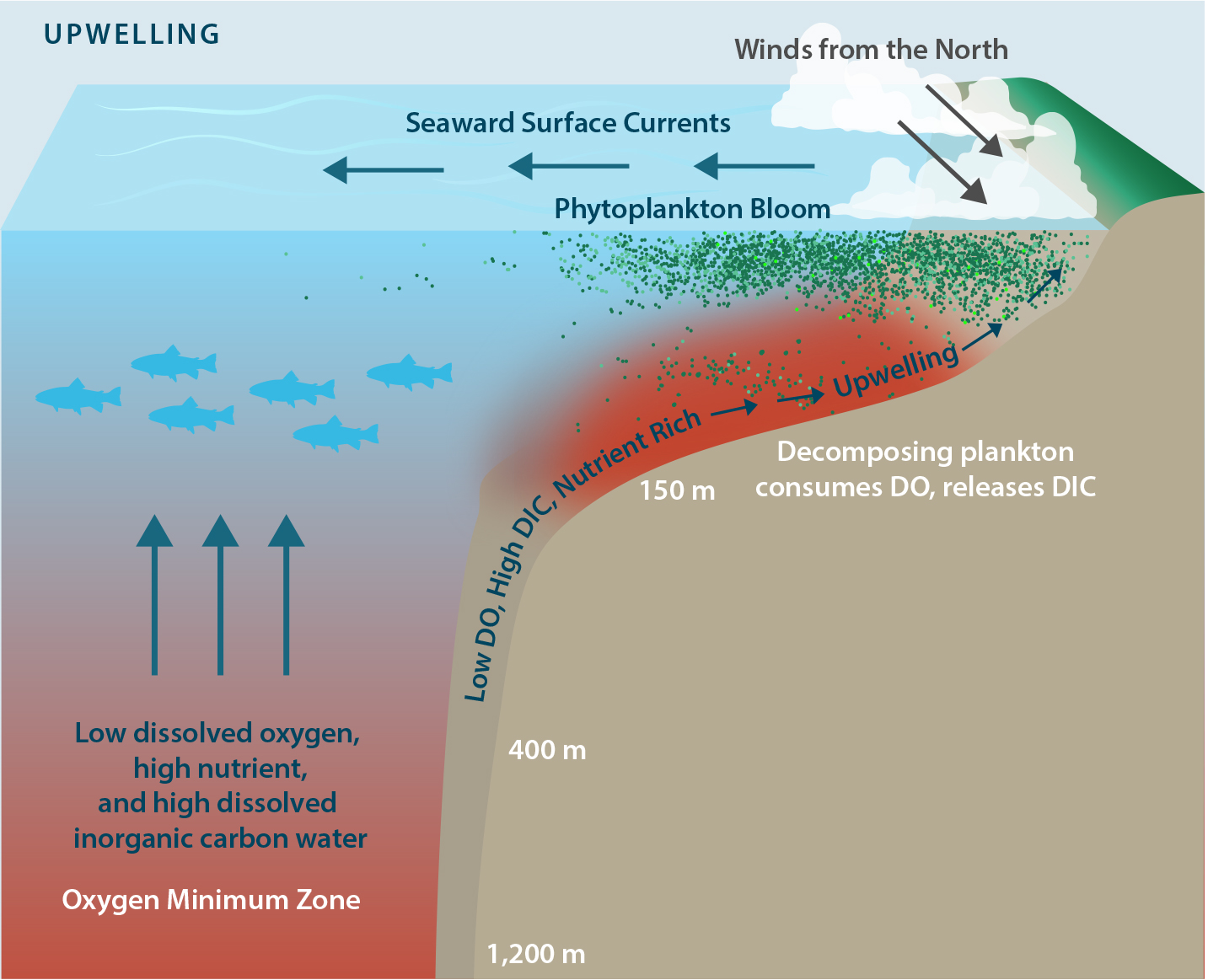
Figure 2. Drivers of hypoxia and ocean acidification intensification in upwelling shelf systems. Equatorward winds drive the upwelling of low dissolved oxygen (DO), high nutrient, and high dissolved inorganic carbon (DIC) water from above the oxygen minimum zone. Cross-shelf gradients in productivity and bottom water residence times drive the strength of DO (DIC) decrease (increase) as water transits across a productive continental shelf. Variations in source water DO, DIC, and nutrient content, upwelling favorable winds, and shelf processes can all accentuate or dampen the severity of hypoxia and OA exposure. Modified from Gewin (2011) by Moni Kovacs. > High res figure
|
The consequences of open-ocean deoxygenation and altered upwelling are of course not confined to the northern CCLME. Low oxygen subsurface waters are also found to varying degrees in each of the other three major upwelling systems around the globe: the Humboldt Current System off Peru and Chile, the Benguela Current System off southwestern Africa, and the Canary Current System off northwest Africa and Iberia. In June 2008, scientists gathered at an international symposium entitled “Eastern Boundary Upwelling Ecosystems: Integrative and Comparative Approaches” in Las Palmas, Gran Canaria, Spain, in the heart of the Canary Current System (Fréon et al., 2009). Engagement with colleagues greatly expanded community understanding of the relative roles of remote vs. local processes in structuring hypoxia formation across the CCLME (Checkley and Barth, 2009) and other eastern boundary current upwelling systems. Partnerships have also been vital in extending research across systems and across disciplines. For example, the discovery of nearshore hypoxia and anoxia in Oregon catalyzed a collaboration between physical oceanographers, biogeochemists, microbial ecologists, and paleoceanographers in Oregon and Chile. The effort provided insights into both the shared dynamics that give rise to upwelling-driven shelf oxygen deficits and the convergent responses of microbial communities to oxygen declines (Bertagnolli and Ulloa, 2017). During 2009–2010, hypoxia was found at between 41% and 79% of bottom trawl locations where profiling DO sensors were available offshore of Washington, and at between 59% and 70% of the stations sampled south of Cape Mendocino, California (44.43°N; Keller et al., 2015). Catch and species richness of fish and shellfish decreased with decreasing near-bottom oxygen concentration. Sustained observations have further enabled modeling studies that are examining sub-kilometer-scale dynamics of DO over large portions of the CCLME. The availability of shelf time series allows for testing and validation of model skill in reproducing and forecasting hypoxia across climate states in the CCLME (Siedlecki et al., 2016; Durski et al., 2017).
Since fishers first reported crab die-offs, our understanding of shelf hypoxia in the CCLME has grown dramatically. For example, we have learned that rather than a one-time localized event (Grantham et al., 2004), hypoxia is a routine, regional-scale, stark expression of an unanticipated climate change impact. PISCO research has played a central role in shaping this understanding and offers lessons in the value of sustaining time-series investigations, growing research networks that can tackle new problems with diverse tools and perspectives, and maintaining flexibility to respond quickly to the novel appearance of unexpected phenomena. In dynamic coastal environments, long-term observations have been vital for capturing anomalous events that reveal drivers as well as realistic trajectories of system changes and impacts. Sustained observations have also yielded insights into system predictability (Figure 1d) and a climatological perspective on interannual dynamism that informs expectations of impacts for fishers and managers.
Ocean Acidification Hits Shore
In the early 2000s, climate scientists warned that ocean acidification was a growing and inevitable problem for the global ocean (Caldeira and Wickett, 2003; Royal Society, 2005). Attention to the vulnerability of the CCLME to OA sharpened greatly with the seminal work of Feely et al. (2008) and the connections it facilitated between OA and shellfish hatchery failure in Oregon (Barton et al., 2012). The very upwelling circulation that fuels the CCLME’s exceptional productivity also brings dissolved inorganic carbon (DIC)-rich waters to shallow coastal waters, making the system highly vulnerable to additional anthropogenic DIC inputs. Resolving ecological vulnerability requires understanding both the level of exposure to acidified water and the sensitivity of the biota to reduced pH and carbonate ion concentration. Thus, studying OA and its impacts created challenges similar to those posed by the rise of hypoxia. Continuous in situ coastal observations of carbonate chemistry were nonexistent, greatly limiting understanding of spatial and temporal variations in acidified conditions and their impacts on biological sensitivities. Uncertainties in ecological risks were further amplified by basic questions about how physiological impacts on individual taxa will scale up to populations and whole food webs, the potential for adaptation to rapid carbonate chemistry changes, and how the effects of OA would interact with already pressing concerns raised by hypoxia. These are questions that remain largely unanswered today.
Because of stoichiometric constraints in the coupled cycling of oxygen and carbon in respiration, remineralization, and photosynthesis in the sea, researchers strongly expected that carbonate chemistry dynamics would reflect those uncovered for DO. Fortunately, the existing PISCO mooring “platform” was well established—collecting time-series data on temperature, salinity, DO, and other measures—and could facilitate new studies of carbonate chemistry. With support from the US National Science Foundation (NSF), PISCO deployed newly available pCO2 and pH sensors to existing moorings in order to empirically characterize the dynamics of OA and hypoxia. Despite differences in rates of gas transfer between oxygen and carbon dioxide, the high-frequency OAH dynamics covaried with surprising strength in inner-shelf waters (Chan et al., 2017). Upwelling events that brought hypoxia to the shore were invariably accompanied by levels of pH and associated aragonite saturation (Ωaragonite) values that have been demonstrated to impair formation of shells in oysters and pteropods (Barton et al., 2012; Bednaršek et al., 2017).
With funding from NSF, PISCO researchers and colleagues with expertise in eco-physiology, evolution, modeling, sensor technologies, and oceanography formed a US west coast research consortium, Ocean Margin Ecosystem Group for Acidification Studies (OMEGAS). Drawing on PISCO’s LME-scale approach to science, OMEGAS sought to quantify and thereby define the seascape of OA exposure across 1,000 km of the CCLME. In addition, the group sought to understand OA impacts in an ecosystem context and evaluate the potential for local adaptation and rapid evolution in response to OA (Hofmann et al., 2014). Through geographically widespread deployments of custom-designed pH sensors that could survive conditions in the surf zone, we discovered that some of the most acidified and dynamic surface ocean exposures could be found in the nearshore. For example, at one Oregon location, seawater pH in the nearshore varied between 7.45 and 8.30 over the course of one day (Chan et al., 2017). Importantly, OA exposure was spatially heterogeneous with temporally persistent, geographically fixed “hot” and “cold spots.”
The discovery of this “mosaic” of OA exposure set the stage for investigations into the scope of biological resilience (see Kroeker et al., 2019, in this issue) to OA as a function of eco-evolutionary dynamics and ecological contexts. Initial studies by the OMEGAS consortium established the potential for adaptive responses among species, and this evolved into the OA exposure mosaic of the CCLME (Hofmann et al., 2014; Evans et al., 2017). Although there was emerging evidence for adaptive responses to OA in short-lived species with rapid turnover times (Lohbeck et al., 2012), a landmark study in the CCLME found that exposure to OA conditions projected for the future had surprisingly little effect on the development of purple sea urchin larvae (Strongylocentrotus purpuratus; Pespeni et al., 2013). Instead, exposure to low pH conditions during larval development selected for tolerant genotypes already existing in the population (Pespeni et al., 2013), with the necessary standing genetic variation for the adaptive response likely driven by the evolution of S. purpuratus in the dynamic pH environment of the CCLME. Additional studies have found evidence of local adaptation in populations from different pH environments in the OA exposure mosaic (Kelly et al., 2013), highlighting the potential for heritability of OA-tolerant genotypes and potential evolutionary rescue, even among long-lived species. Continuing research on the locally adapted populations of purple sea urchins has opened up new questions about the long-term fitness consequences of the mechanisms underlying tolerance to OA, which involves upregulating energetically costly metabolic pathways to maintain homeostasis (Evans et al., 2017).
There is growing recognition that OA is one of many factors that organisms will have to cope with in the future, especially in dynamic eastern boundary upwelling systems (Bopp et al., 2013). The environmental mosaic provided an opportunity to test how different factors influence the ecological effects of low pH exposure. Due to the links between OA and upwelling in the CCLME, low pH exposure of nearshore, coastal species co-occurs with high seawater nutrient concentrations. Nutrient infusions into the photic zone can promote exceptional nearshore phytoplankton blooms. However, this supply of nutrients is not homogeneous in space, and differences in currents that flush versus retain phytoplankton cells nearshore can lead to wide differences in food available to benthic organisms. Studies spanning the OA exposure mosaic found that the effect of low pH exposure on the growth of the California mussel (Mytilus californianus) was dependent on these algal blooms as a source of food. When conditions facilitated phytoplankton blooms, we found that mussels were able to maintain high growth rates at sites with frequent low pH exposure. In contrast, mussels grew the slowest in persistent upwelling sites, with frequent low pH exposure but limited productivity (Kroeker et al., 2016). These studies set the stage for putting OA effects into a broader ecosystem context in which multiple drivers and interactions among species create geographic variability in the potential effects. The science of resilience and its intersection with management is discussed in Kroeker et al. (2019, in this issue).
Science Informing Policy
The growth in OAH science in the CCLME has been accompanied by rising attention from policymakers. As a result of PISCO and NOAA scientists’ briefings of key policymakers in Washington State, the governor of Washington established a Blue Ribbon Panel on OA. Following reports from the Washington State Blue Ribbon Panel (WSBRPOA, 2012; Feely et al., 2012), the governors of the three US west coast states and the premier of British Columbia convened “The West Coast Ocean Acidification and Hypoxia Science Panel” in 2013 to summarize major findings on OAH for the North American west coast and to make specific recommendations for dealing with ocean acidification and hypoxia (Chan et al., 2016). By 2017, the three US west coast states along the CCLME had enacted legislation to address the challenges of OA or OAH. The legislation was state-specific, but each state established a formal advisory body and processes for science to inform policy and management actions to address ocean chemistry changes. Washington, Oregon, and California are also founding members of The International Alliance to Combat Ocean Acidification, which promotes the development and implementation of OA Action Plans to guide national, municipal, and organizational priorities and responses.
The importance of cross-jurisdictional partnerships can also be seen in a recently completed joint effort between the Pacific Coast Collaborative, which is made up of the governors of the three West Coast states, the governor of Alaska, the Premier of British Columbia, and the US Federal Interagency Working Group on Ocean Acidification. By building on such innovative industry, academia, and agency collaborations such as the California Current Acidification Network (C-CAN), this new partnership is providing the first comprehensive inventory of OAH biogeochemical and biological monitoring assets across the Northeast Pacific. The goal is to telescope in from global efforts such as the Global Ocean Acidification Observing Network (GOA-ON) to provide local and regional decision-makers with a clear understanding of where gaps in OAH observations may lie relative to management decisions that are within their jurisdictional purview (Figure 3).
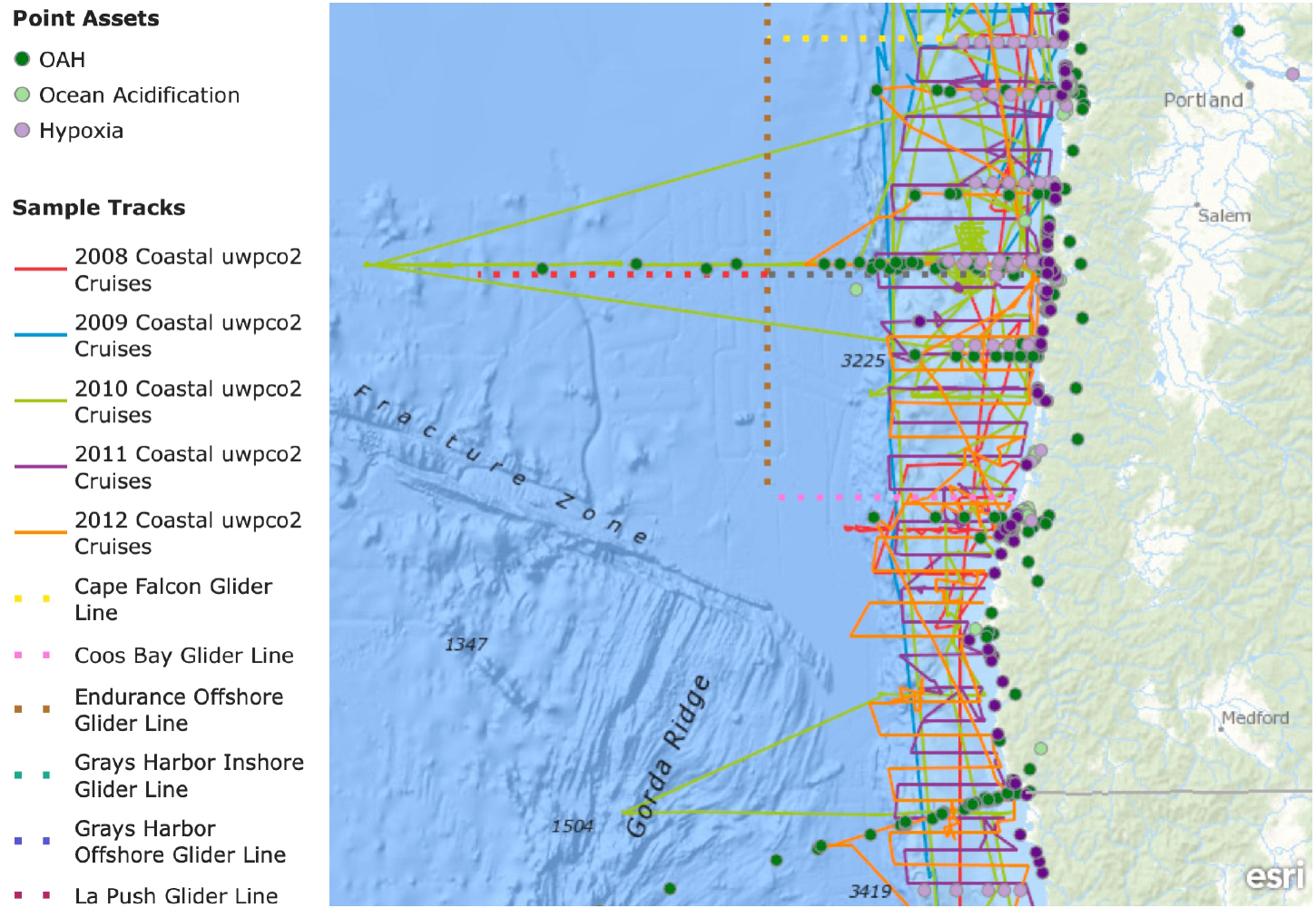
Figure 3. Ocean observations for hypoxia and OA along the Oregon coast as compiled by the West Coast OAH (ocean acidification and hypoxia) Monitoring Inventory project (https://oceanmonitoring.cnra.ca.gov/). In 2002, observing assets were restricted to ship-based DO measurements by PISCO and GLOBEC (Global Ocean Ecosystem Dynamics) researchers. Present-day observing aspects in the region include repeat ship-based surveys for DO and carbonate chemistry parameters, mooring-based sensors, and autonomous underwater vehicle-based DO measurements, as well as DO observations integrated with fishery surveys. Point assets refer to the locations of moored instruments or ship-based sampling stations (vs. underway surface measurements) and are color-coded to reflect whether measurements involve DO, carbonate chemistry (OA), or both (OAH). It is important to note that only a subset of the observations have monitored DO or carbonate chemistry continuously at depth, and a much smaller subset of assets are continuing to do so. > High res figure
|
Another example of science informing policy is in the March 2019 US Environmental Protection Agency authorization regarding discharge of seafood processing waste under the National Pollutant Discharge Elimination System for “Offshore Seafood Processors Discharging in Federal Water off the Washington and Oregon Coast” (USEPA, 2019). With knowledge of the spatial distribution and temporal behavior of near-bottom hypoxic waters off central Oregon based on dissolved oxygen measurements from ships and moorings as described here, scientists contributed knowledge to inform the creation of an exclusion zone to the discharge permit. The year-round exclusion zone extends as far as 36 nautical miles offshore and encompasses the Heceta/Stonewall Bank complex.
From initial ad hoc ship-based measurements to a coast-wide network of ship, mooring, and autonomous vehicle-based sensors, OAH observations in the CCLME have advanced considerably (Figure 3). Similarly, from the first reports from the commercial crab fleet and oyster growers to enactment of OAH legislations, the policy response across the CCLME has been remarkably rapid (see Lubchenco et al., 2019, in this issue). What lessons can we draw from the past decade and a half of research? First, the ability to sustain observations has been essential. By establishing an in situ, nearshore DO time series where none existed previously, the research and management community had access to data that captured the sometimes ephemeral seasonal onset of severe hypoxia or anoxia. This information defined the scope of the problem, described its variability in space and time, and revealed the connections between nearshore hypoxia and remote changes in coastal winds and deep ocean oxygen content. Over time, sustained observations also permitted us to share information when a hypoxic event was especially intense or persistent. Knowing the strength of exposure anomalies in turn facilitated interpretation of biological anomalies that were observed by fishers, researchers, and managers.
Second, the importance of forging observational partnerships cannot be overstated. The PISCO moorings anchored a coast-wide network for detecting and tracking the onset of OAH events and their impacts. This meant having a broad suite of partners who trained a diverse set of eyes on the ocean to expand our understanding of the geographic scope and depth of biological impacts of hypoxic events. This network continues to grow. In Oregon, a partnership between academic scientists, state and federal agencies, industries, nongovernmental organizations, and tribal representatives has formed to monitor DO, carbonate chemistry, and biological changes at the state-wide scale. In Oregon (Box 3) and California, PISCO scientists are working with citizen scientists to monitor pH in marine reserves. Such partnerships greatly enhance the understanding of OA in habitats of key management concern that would otherwise go unmonitored for OAH. In retrospect, the funding support that allowed PISCO to respond quickly, monitor change, and sustain research and outreach on emerging environmental changes has been transformational. Importantly, demands on science to inform new policy and management challenges will only grow with global change. While there are always opportunity costs to committing to long-term investigations, PISCO’s experience with OAH hopefully offers insights into the value of catalyzing research and partnerships to inform society’s decisions.
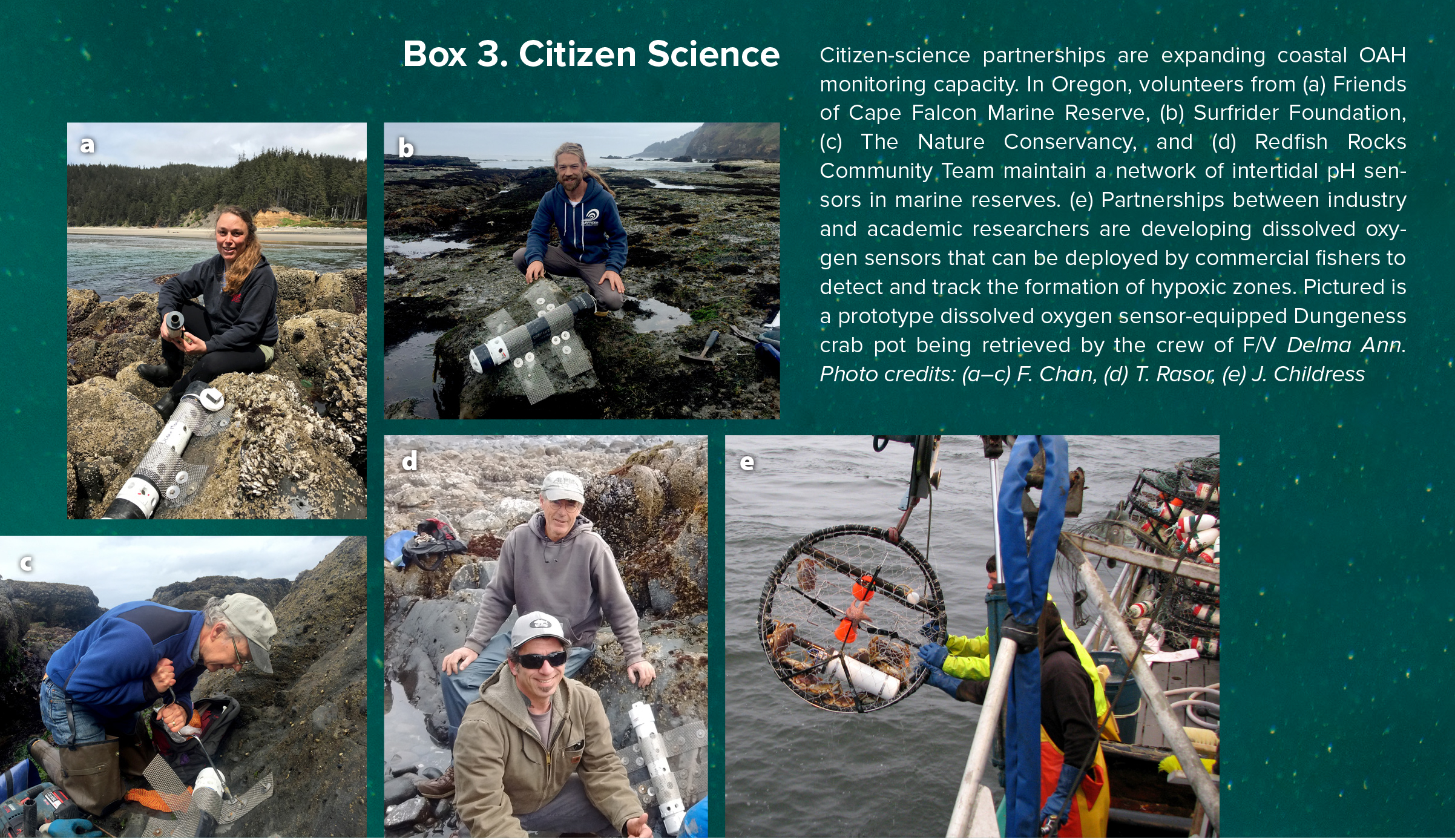
|
The combined expression of OAH exemplifies the evolving approach to global change research from a single stressor (e.g., temperature) to a multi-stressor and temporally and spatially variable exposure challenge. Over time, OAH research has sharpened in focus from issues of uncertain impacts to direct concerns for management of fisheries, marine protected areas, coastal water quality, land use, and habitat conservation (Boehm et al., 2015; Weisberg et al. 2016). In parallel, public and decision-maker needs have evolved from one of issue identification to demands for mitigation and adaptation actions. These rapid shifts will also bring new challenges for science. As we move into the second decade of integrated OAH research, science will need to provide a fuller, and often system-specific, assessment of risks if society is to have the information necessary to take actions that are not only proactive but also prioritized, given available resources.
The net effects of anthropogenic carbon emissions on upwelling ecosystems remain challenging to predict. Coastal upwelling winds are difficult to fully capture in global Earth system models (Di Lorenzo, 2015), and increased upwelling winds may increase nutrient supply (Jacox et al., 2015) but push primary production offshore (Ruzika et al., 2016), making the coastal expression of hypoxia and ocean acidification more difficult to forecast. In addition, rather than a general CCLME-scale understanding of exposure, stakeholders and managers are already requesting information on current and future OAH exposure on a port-by-port, or marine reserve-by-marine reserve, basis. At the same time, new knowledge of biological sensitivity in response to realistic, dynamic exposure regimes will be needed. This will require new understanding of how sensitivity scales up to population, community, ecosystem, and social impacts, and to the capacity for organismal to ecosystem-scale resilience if we are to achieve an accurate and decision-relevant picture of vulnerability. This transition toward solution building and resilience management will further require knowledge of how different management decisions intersect with ecological and social vulnerability, whether decisions support or erode resilience, what meaningful local mitigation and adaptation solutions can be employed, and what are the trade-offs, interactions, and effectiveness of these solutions over time. These are not trivial challenges—they will require a strategic approach to research that can deliver decision-relevant answers.
Acknowledgments
We thank the many undergraduate students, graduate students, research assistants, postdoctoral scholars, and collaborators who have contributed to our continually expanding understanding of California Current System dynamics. Besides support by the David and Lucile Packard Foundation, the Gordon and Betty Moore Foundation, and the Andrew W. Mellon Foundation, research was supported by Oregon Sea Grant, the National Science Foundation, and the National Oceanic and Atmospheric Administration. This is PISCO publication number 496.