Introduction
Interest in the physics, chemistry, and potential for life on other planets has been widespread among the general scientific community, and this interest has been increasing in recent years. A number of missions have either been completed (e.g., Galileo, Voyager, Cassini-Huygens) or are in the planning phases (e.g., Europa Clipper, Europa Lander, Dragonfly) to characterize the conditions of some of the outer planets and their moons and to determine whether they harbor conditions that could be suitable for life. The most promising of these are ocean worlds within our own solar system, such as the moons of Saturn (Enceladus), Jupiter (Europa), and Neptune (Triton) that are covered in liquid water beneath a thick layer of ice. Internal heat sources prevent this liquid water on the lunar surfaces from freezing, possibly providing the conditions necessary for the evolution and sustenance of microbial life (Lobo et al., 2021).
The cost and long time horizons needed to plan and mount missions to study these ocean worlds means that very few missions are possible. To maximize the probability of detecting life on ocean worlds, it is necessary to first understand the suite of conditions required to support life on Earth and use this information to search for similar or analogous habitats on other ocean worlds. To do so, researchers seek out extreme environments on Earth where the physical and chemical conditions may result in an environment that is a reasonably close analog to habitats on other worlds. The most likely analog environments are found either near the poles, where kilometers thick ice sheets, isolated saline lakes, and seasonally forming sea ice provide unique but extreme habitats for microbial communities here on Earth (Garcia-Lopez and Cid, 2017), or in the deep sea where tectonic activity produces energy-rich hydrothermal plumes that support an entirely chemosynthetic food web (Michalski et al., 2017) (Figure 1).
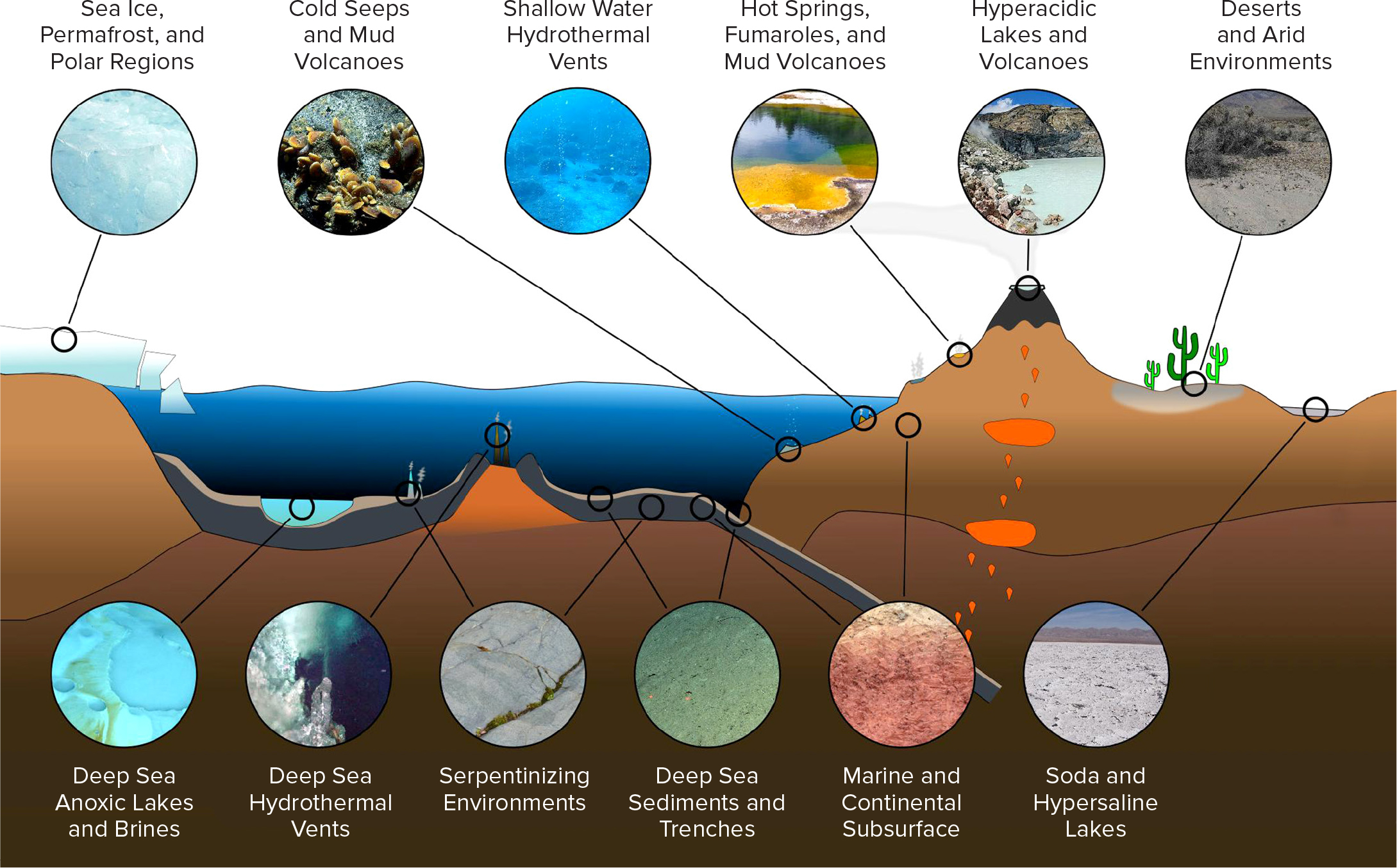
FIGURE 1. Representative idealized cross section of Earth’s crust showing the diversity of extreme environments where microbes have been found and their approximate location. Figure modified from Merino et al. (2019). > High res figure
|
Analog environments on Earth enable us to begin to answer research questions about extraterrestrial ocean worlds that would be otherwise inaccessible. In addition, because of their relative ease of sampling and much closer proximity to research facilities (compared to lunar landing missions), utilization of analog environments allows scientists to undertake many investigations for the cost of a single planetary mission. This ability for rapid testing of sampling techniques and experimentation on analog environments would provide an effective test bed for the kind of operations required for future ocean world missions and increase the likelihood of mission success. For example, before we can drill through and sample the ocean beneath the moon Europa, where the ice is much colder than glacial ice on Earth, we need to demonstrate an ability to remotely drill through the ice sheets of Antarctica or Greenland. To this end, it may be useful to consider how the ice and underlying water interact in areas such as the under-ice shelf environment or double-diffusive convection beneath sea ice.
How Can We Use Models to Inform Scalability, Sampling, and Operations for Analog Environments?
In addition to direct in situ sampling, different modeling approaches can be useful and cost-effective tools both for determining whether another planet or its moon is indeed a suitable Earth analog and for designing strategies to sample extraterrestrial analog environments. While even the most sophisticated Earth system models are gross oversimplifications of reality, they can still provide useful information. For example, reconfigured Earth system models like ROCKE-3D that runs on NASA’s Discover supercomputer (Aleinov et al., 2019) can be used to determine if conditions on other ocean worlds are suitable to support life. Additionally, the Virtual Planetary Laboratory at the University of Washington develops and combines a variety of scientific models from many disciplines and with varying degrees of complexity to constrain habitability for newly discovered worlds. These models could be used to study exoplanets or other ocean worlds within our own solar system.
Slightly more complex hierarchical modeling frameworks allow users to model a planetary system at different levels of complexity, based on the underlying characteristics of the system under investigation. One such model (Isca) has been used to model planets such as Earth, Mars, and Jupiter at different resolutions and with a different suite of processes (Thomson and Vallis, 2019). More targeted models, such as the Titan Community Atmosphere Model (CAM), have been used to better understand highly specific processes such as the dune-forming wind fields on this large moon and how they are impacted by topography and torque (Larson, 2019).
Once models show that a particular ocean world has conditions suitable to support life, they can be used to test our understanding of the underlying physics, which will allow us to design better sampling strategies for analog environments, both here on Earth and on extraterrestrial ocean worlds. For example, ice sheet models (Farinotti et al., 2021) developed for Greenland and Antarctica can help target the most suitable locations for drilling and subsequent sampling. All ice sheets flow, albeit slowly—and in some instances very slowly—and this motion together with any associated horizontal shear needs to be accounted for when designing a sampling strategy, particularly for portions of the ice sheet that have not yet reached the ocean. Ice sheet models can provide valuable information about vertical stress gradients (due to differences in horizontal flow speeds at different depths within the ice) as well as temperature gradients (which control ice deformation) for an ice sheet of interest, aiding in the determination of appropriate sampling sites.
Models can also be used to scale field observations and site-specific measurements to those spatial scales that are relevant for the space-based explorations that rely on either satellite-based or lander-based measurements (Schinder et al., 2011). In situ sampling of extreme environments on Earth is often a logistically difficult and expensive endeavor, resulting in collection of relatively few samples over fairly restricted time horizons. The use of three-dimensional models allows us to synthesize these measurements into an appropriate geophysical framework, and to scale them up with respect to both space and time, so that we can gain a broader perspective of the analog environment of interest. Using models in this way allows us to convert static point measurements into time-dependent processes in multiple physical dimensions, resulting in a fuller understanding of the underlying physical processes.
Both Earth and planetary scientists are critical to the development of appropriate analog models. Planetary models of chemistry and physics are based on models developed first for use in simulating processes on the Earth. Models of atmospheric dynamics, hydrothermal vent systems, ice sheet dynamics and thermodynamics, and sub-ice ocean interiors developed by Earth scientists are being used by planetary scientists as a basis for developing models for extraterrestrial ocean worlds. This is possible because many of the equations governing the fundamental physical and chemical processes here on Earth are the same as, or very similar to, those on other ocean worlds, so the models often only require simple adjustments to a few key parameters. For example, general circulation models (GCMs) developed to describe atmospheric dynamics on Earth have been modified for use on other bodies in the solar system such as Mars (Wilson and Hamilton, 1996; Forget et al., 1999; Rafkin et al., 2001; Richardson et al., 2007), Venus (Richardson et al., 2007), and Titan (Richardson et al., 2007; Lora et al., 2019). One such model that has proven especially useful for this purpose is the Weather Research and Forecasting (WRF) model, which was developed collaboratively by National Center for Atmospheric Research, the National Oceanographic and Atmospheric Administration, the US Department of Defense, and a number of research universities (Michalakes et al., 2004; Skamarock et al., 2005). This model, and others like it, can be run in one, two, or three dimensions and at resolutions ranging from meters to kilometers. Thus, Earth scientists have an important role to play in providing many of the basic model frameworks for describing processes such as ocean and ice thermodynamics, fluid flow, and key chemical processes that can then be modified for use on other ocean worlds. Earth scientists will also be required to identify those analog environments on Earth that are amenable to modeling and where lessons learned there can provide critical information and insights for developing analogous models for extraterrestrial ocean worlds.
The planetary science community can contribute the theoretical frameworks needed to translate Earth system models to models of extraterrestrial ocean worlds, as well as critical observational data from missions such as Cassini-Huygens to constrain those models (Tokano, 2009, 2013, 2019). Atmospheric dynamics such as cloud formation and precipitation can be detected using the suite of sensors like those on Cassini-Huygens (Turtle et al., 2018), as can surface topography (e.g., mountains, canyons, lake beds, channels, and dunes). This greater data quantity and quality from Titan has inspired planetary scientists to develop numerous GCMs of Titan’s climate system. These models allow planetary scientists to infer atmospheric processes such superrotation, circulation and chemistry of the middle atmosphere, tropospheric methane cycling, interactions of surface and atmosphere, and even Titan’s paleoclimate (Lora et al., 2019). Because many of these modeling studies are largely independent, the planetary modeling community has increasingly relied on multi-model results and model intercomparison projects (Lora et al., 2019) to understand and account for potential model biases and structural differences to ensure that results are not model-dependent.
How Can Work at Analog Environments Inform Planetary Protection Strategies/Needs?
The advent of space exploration has made the protection of Earth’s environment and other solar system bodies from harmful contamination an important principle of high priority. From 1958 onward, the development and implementation of planetary protection policies has taken place at both the national and international levels. Scientific advances have permitted the targeting of these policies to those few bodies thought to be capable of harboring life (extinct or extant), or processes relevant to prebiotic chemistry. This trend in increasingly targeted planetary protection is expected to continue as the exploration of ocean worlds of the outer solar system progresses; this is important given that, by one estimate, planetary protection accounts for approximately 1% (for lander missions not carrying instruments for the investigation of extant life) to 5% (for lander missions designed to investigate extant life) of the cost of a typical mission (Bearden and Mahr, 2017).
Generally speaking, planetary protection policies address all missions to and from all types of solar system bodies (planets, moons, comets, and asteroids) and places those missions into categories based on mission type, the likelihood of that target harboring life, and the probability that either organisms brought from Earth could survive on that body (forward contamination) or that material returned to Earth would pose a risk here (back contamination). However, based on existing knowledge and capabilities, only three bodies are currently of significant planetary protection concern: Mars, Europa, and Enceladus (NASEM, 2018). Of course, this may change as we learn more about the moons of the outer planets in our solar system.
Recent biological discoveries in analog environments on Earth related to extremophiles, biofilms, prions, and genomics are likely to be relevant to planetary protection science. Metagenomic analyses of organisms living in extreme environments on Earth (e.g., dry polar or desert environments, ice sheets, hydrothermal vents, oceans, anoxic zones) generate lists of archaeal and bacterial species and specific genes that are necessary to thrive in environments that are the closest analogues of other ocean worlds in our solar system. This type of research facilitates a more targeted approach to planetary protection. Rather than treating all microbial species as potential contaminants of extraterrestrial ocean worlds, it might be possible to target contamination controls on just those microbes that are found in analog Earth environments that are most like the landing sites on icy ocean worlds, and to survey spacecraft assembly rooms for those organisms (Shtarkman et al., 2013; Abreu et al., 2016). In this way, information about the abundances of specific microbes with known environmental tolerances could increase our ability to implement a probabilistic approach to planetary protection for missions to the outer planets and their icy moons (DiNicola et al., 2018).
Currently, the icy body contamination requirement is that the probability of inadvertent contamination of an ocean or other liquid water body must be less than 1 × 10−4 per mission (NRC, 2000, 2011; Sherwood et al., 2017; Lamy et al., 2018; COSPAR, 2020). Biological contamination is defined as “unwanted presence of biologically produced molecules carried by spacecraft hardware, including instruments and experiments, that compromise understanding of extraterrestrial environments” (NASA Procedural Requirement [NPR] 8715.24). The instruments to be used on robotic missions to ocean worlds are decontaminated using a variety of methods (Meltzer, 2011), including treatment by radiation (e.g., gamma radiation), chemicals (e.g., hydrogen peroxide), high temperature sterilization (e.g., autoclaving and dry heat), and lower temperature sterilization (e.g., hydrogen peroxide plasma). It must be noted, however, that the application of these methods may not be completely effective. For example, hydrogen peroxide vapor sterilization does not impact organisms within enclosed volumes on spacecraft or enclosed within other materials. In addition, some extremophiles have been shown to exhibit radiation hardiness to the levels used for decontamination (5.5 Mrad) (NASEM, 2018). Thus, the study of organisms from analog environments on Earth could provide for more robust testing of decontamination procedures, thereby increasing their effectiveness.
The development of planetary protection policy in the United States and internationally preceded the 1967 adoption of the Outer Space Treaty (OST). Article IX in the OST states that all parties “shall pursue studies of outer space, including the Moon and other celestial bodies, and conduct exploration of them so as to avoid their harmful contamination, and also adverse changes in the environment of the Earth resulting from the introduction of extraterrestrial matter.” As of 2018, the OST had been ratified by 105 countries (and signed by 25 others), including all of the spacefaring nations. Technical aspects of planetary protection have been developed internationally by the Committee on Space Research (COSPAR), which is part of the International Council for Science (ICSU) and is consultative with the United Nations Committee on the Peaceful Uses of Outer Space (COPUOS). COSPAR is a scientific organization whose purpose is to “provide the world scientific community with the means whereby it may exploit the possibilities of satellites and space probes of all kinds for scientific purposes and exchange the resulting data on a co-operative basis.” As per COPUOS, it has been an effective forum for the development of international consensus on planetary protection guidance for science exploration missions (NASEM, 2018).
To achieve this consensus, government organizations such as NASA may formulate and implement planetary protection policies and procedures to be consistent with COSPAR’s planetary protection policy. To do so, NASA gathers scientific input from the Space Studies Board (SSB) of the National Academies of Sciences, Engineering, and Medicine, agency advisory groups, and consultants to the Office of Planetary Protection (OPP). US law lacks a clear regulatory pathway to apply the OST to private-sector spaceflight activities beyond launch, landing, and communications approvals, although in Article VI of the OST, it is clear that nongovernmental activities fall within that scope, for example, “States Parties to the Treaty shall bear international responsibility for national activities in outer space, including the moon and other celestial bodies, whether such activities are carried on by governmental agencies or by non-governmental entities.” Article IX, which establishes the need for planetary protection, requires international consultations for activities by a signatory state “or its nationals in outer space” that would potentially interfere with other activities. Given the increasing activity of the private sector in developing and launching space missions in recent years, it will be necessary to find ways to regulate and monitor their plans for implementing effective planetary protection protocols (NASEM, 2018).
It can be said that effective planetary protection implementation depends on identification of the thresholds for the survivability of microbes and development of methodologies to eliminate them. Therefore, both the space science and Earth science communities can contribute to the development of policies, procedures, and techniques to meet planetary protection requirements, and both communities can contribute to testing possible solutions in analog field sites.
In recent years, NASA’s planetary exploration priorities have evolved in three directions: (1) placing special emphasis on robotic exploration of ocean worlds (relevant to ocean worlds), (2) developing an effective plan for sample return missions classified as “restricted Earth return” (which may become relevant to ocean worlds, especially Enceladus), and (3) developing protocols to enable the human exploration of Mars. In light of these priorities, modern biology, specifically the ability to sequence the genomes of hundreds of thousands of organisms, offers a scientific pathway to future development of planetary protection policy that would benefit from the participation of both Earth and planetary scientists.
To make use of their expertise and experience, scientists affiliated with the NASA Astrobiology Institute, who have been active in studies relating to extremophile microbes on Earth, what sorts of biochemistry they use, and the origin of life, should become more substantively and directly involved in the planetary protection process. This participation should be reflective of the recent recommendations by the National Academies that studies, workshops, and brainstorming sessions organized by NASA and other space agencies (e.g., ESA and JAXA), as well as the SSB and COSPAR, should include a sufficiently broad range of microbiologists (NASEM, 2018) to enable the development and implementation of more effective partnerships among Earth scientists and planetary scientists capable of testing planetary protection solutions at field sites.
“Utilizing analog environments on Earth can be an effective way to further conceptualize, develop, and parameterize models that may be suitable for extraterrestrial ocean worlds.”
|
Conclusion
The study of analog environments on Earth requires collaboration among different scientific communities, each with their own specific disciplinary expertise. Members of the planetary science community need to identify those physical, chemical, and biological processes on extraterrestrial ocean worlds that remain poorly understood and require further study. For example, the thickness of the ice shells on moons such as Europa and Enceladus and the composition of the water below are not well known and require the identification of technologies and approaches efficacious for narrowing the range of important parameters (e.g., salinity, temperature, chemical makeup). Using what information is available for specific ocean worlds, members of the Earth science community would then be well positioned to identify analogous environments on Earth where these technologies and approaches can be rigorously tested at a reasonably low investment of time and money (which may also prove useful in terrestrial environments on Earth). These analog environments would also provide technologists with the requisite information needed to develop suitable sensors and sampling platforms for measuring and observing the identified physical, chemical, and biological processes.
Utilizing analog environments on Earth can be an effective way to further conceptualize, develop, and parameterize models that may be suitable for extraterrestrial ocean worlds. The first step will be for Earth and planetary scientists to agree on which environments on our planet represent suitable analogs for extraterrestrial environments. Once those decisions are made, models of the analog environments can either be developed or, if possible, existing models can be refined, to simulate the critical processes driving them. Initial development and testing of these models for Earth environments is necessary because if models are unable to simulate the critical processes driving analog environments here on Earth, they are unlikely to be effective tools to understand other ocean worlds. Once a model works well for a particular Earth environment, the next step would be for Earth and planetary scientists to determine what modifications need to be made to the model to make it suitable for simulation of other ocean worlds. After these modifications have been implemented and the model rigorously tested against available observational data from an extraterrestrial ocean world, the model can be used for a variety of purposes on other ocean worlds, including testing our understanding of critical processes, identifying appropriate sampling strategies and locations, and determining which parameters may be the most important for designing appropriate sensors.