Motivating Science Questions
Many of the science questions driving ocean worlds exploration are framed in the context of understanding Earth’s ocean and cryosphere, their biogeochemical cycles, and their physical properties. However, the unique chemistry and environment of each ocean world raises science questions that are specific to each planetary body. For example, on Jupiter’s moon Europa, there is good evidence that there is a slightly salty global ocean, potentially larger than Earth’s ocean. Here, are there organics in the ice or ocean? Do plumes of water ice originate from that ocean? On Saturn’s moon Enceladus, there is evidence of a plume at the southern pole containing organics and that the plume likely originates from that global ocean. Are there biomarkers in the plume that may permit direct in situ orbital sampling? On Titan, could the unique cycling of hydrocarbon clouds, rain, rivers, and lakes on the surface serve as a medium to support life? Can we observe the benthic surface beneath the waves? Indirect evidence exists for a subsurface ocean—does this permit upwelling of material to the surface? Finally, for each of these moons, what redox couples are available to support life, and what is the history of the ocean? These and other ocean world science questions could be addressed in part by the technologies discussed next.
Orbital Technologies
Orbital platforms have thus far provided the majority of knowledge of ocean worlds with such missions as Aqua/MODIS, Landsat, ICESat, and Sentinel on Earth, and Voyager, Galileo, Juno, and New Horizons for the rest of the solar system (Figure 1). Here we provide an overview of the core remote-sensing technologies and developments that may be used on upcoming orbital missions for ocean worlds. Next-generation remote-sensing instruments require advances in both passive and active sensing technologies in order to compare to some of the most precise sensors that already exist in the ocean.
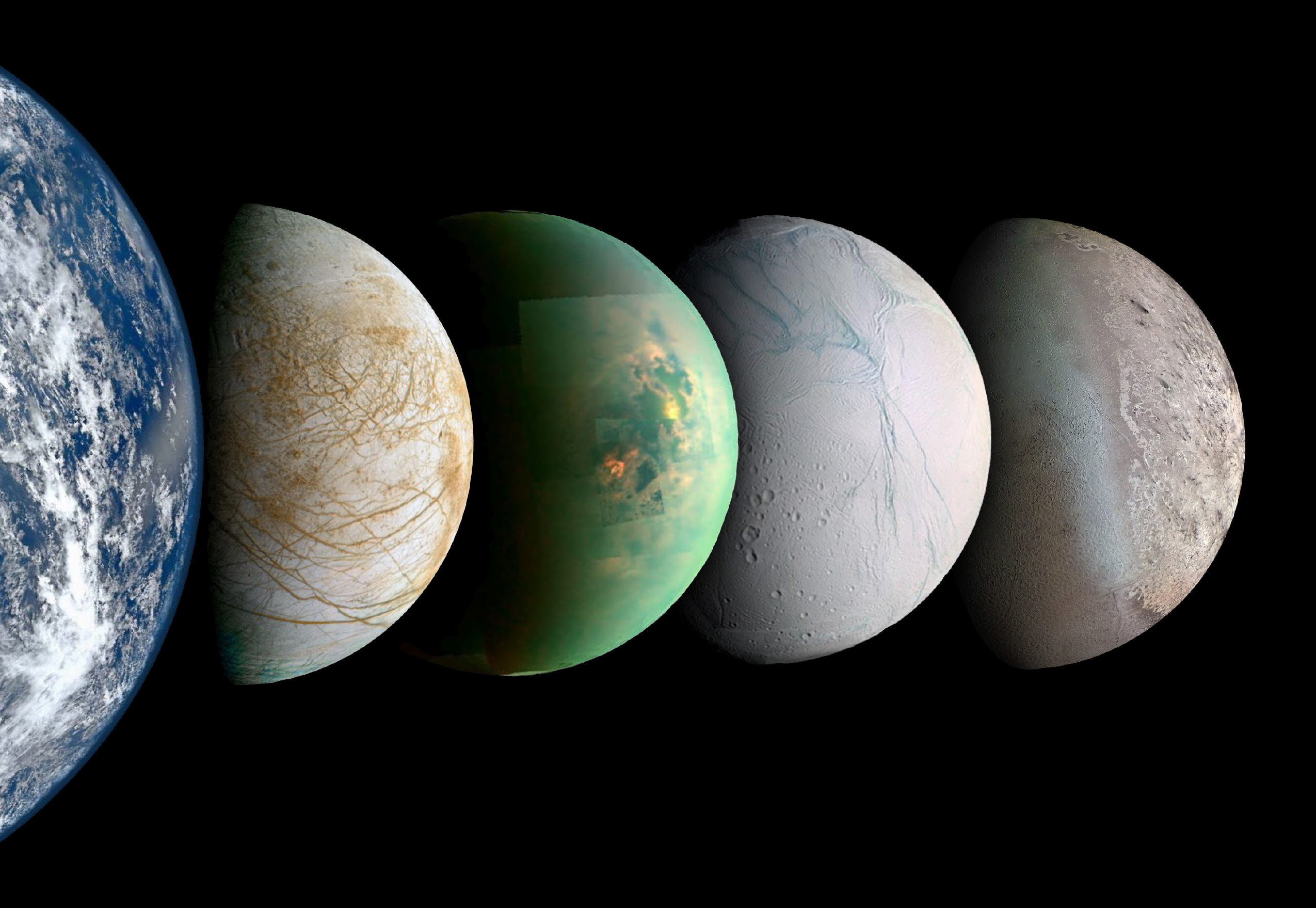
FIGURE 1. Oceans Across the Solar System. From left to right: Earth, Europa, Titan (infrared band), Enceladus, and Triton. Images not to scale. Image credits: Earth – NASA/NOAA/GSFC/Suomi NPP/VIIRS/Norman Kuring; Europa – NASA/JPL-Caltech/SETI Institute; Titan – NASA/JPL-Caltech/University of Arizona/University of Idaho; Enceladus – NASA/JPL-Caltech/Space Science Institute; Triton – NASA/JPL/USGS. > High res figure
|
Passive Optical Remote Sensing (Using the Sun as a Source of Illumination)
Remote sensing and multispectral imaging of environments from air and space primarily use passive broad-spectrum illumination provided by the sun coupled with sensitive push-broom sensor line array photodetectors fitted with narrowband filters to produce multispectral images (Irons et al., 2012). Hyperspectral remote sensing extends this concept by using photodetectors and scanning spectrometers to resolve hundreds or even thousands of spectral bands (Eismann, 2012). However, in both techniques, atmospheric conditions and the distribution of solar radiation limit the frequencies of light and the signal-to-noise ratios (SNRs) attainable for multispectral imaging on Earth and beyond. SNR can be increased by a larger light gathering optic, such as a larger telescope, or by increased pixel size and sensitivity. In addition, atmospheric corrections are routinely applied to Earth-based remote sensing, and similar algorithms would need to be developed for alien atmospheres such as the Saturn moon Titan’s substantial atmosphere.
Airborne and spaceborne sensor technology has advanced rapidly in the last few decades to include imaging spectrometers that span ultraviolet to infrared wavelengths. Because aquatic systems strongly absorb light, dedicated sensors with large dynamic ranges and longer integration times are often required to achieve the sensitivity needed for aquatic applications (Mouroulis et al., 2008). In addition, refractive distortions and the presence of ocean waves can severely impact the effective resolution and SNR of an image. In aquatic systems, further bounds on optical remote sensing are introduced as only ultraviolet and visible bands of light penetrate the photic zone (the top 100 m). As such, current passive multispectral/hyperspectral imagers are limited by ambient conditions along the optical path, the ambient illumination spectrum, the optical aperture, the photodetector SNR, and, consequently, relatively long integration times.
Recently, NASA developed the FluidCam instrument and fluid lensing technology for enhanced imaging of underwater objects. These technologies capitalize on refractive distortions from surface waves that magnify optical lensing elements, or fluid lensing lenslets, to enhance the effective spatial resolution and signal-to-noise properties of remotely sensed images to create new three-dimensional images at the centimeter scale (Figure 2; Chirayath and Earle, 2016). Fluid lensing has applications for remote sensing of ocean worlds with varying fluid interfaces, such as the hydrocarbon seas on Titan.
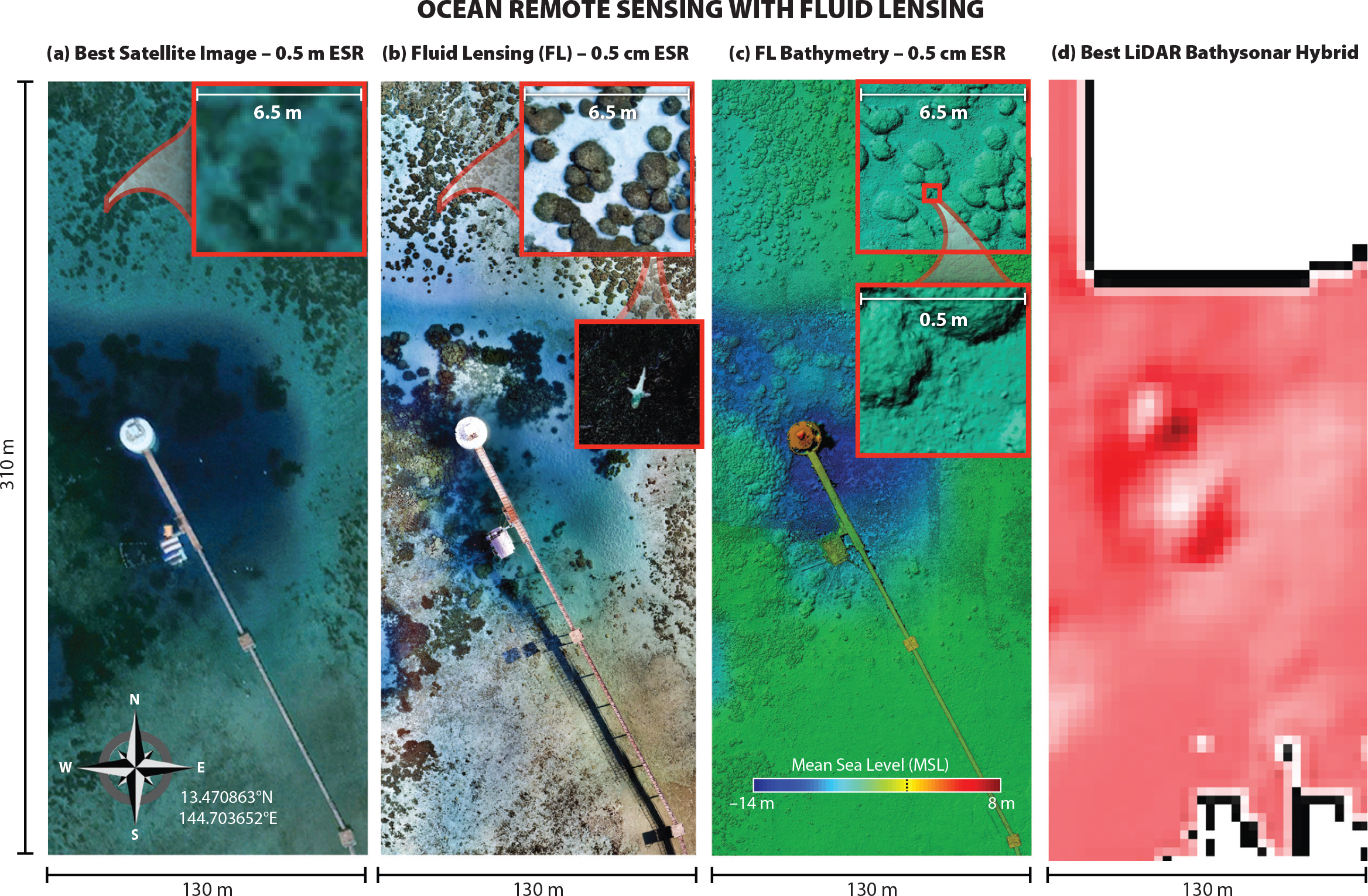
FIGURE 2. Next-generation ocean remote sensing with fluid lensing. Novel technologies such as fluid lensing permit multispectral three-dimensional benthic imaging without ocean wave distortion and caustic noise at increased sign-to-noise ratio, effective spatial resolution (ESR), and depth. Sample data set from airborne Guam 2021 campaign. Fluid lensing can be modified to work in different fluid environments, such as hydrocarbon seas on Titan, or applied to imaging through refractive distortions, as may be present near active deep sea hydrothermal vents on oceans across the solar system. Panels a–c from airborne Guam 2021 campaign (Chirayath, 2021). Panel d from NOAA Pacific Islands Fisheries Science Center, and JIMAR, University of Hawaii. > High res figure
|
Hyperspectral imagery contains a “spectral fingerprint” that can allow assessment of the composition of inorganic and organic matter in the water or within snow and ice. Extensive hyperspectral algorithms are available for characterizing properties of snow, including crystal size and radiative forcing by impurities. Hyperspectral data can also be used to detect different types of light-absorbing compounds, such as ancillary pigments in microalgae and different types of colored dissolved organic matter (e.g., humic materials) that are associated with algal life on snow or ice and in the ocean (Bracher et al., 2017). For example, major groups of phytoplankton can be differentiated in the global ocean (cyanobacteria, diatoms/dinoflagellates, haptophytes, and green algae) by diagnostic biomarker pigments that absorb different increments of the visible spectrum (Kramer and Siegel, 2019). Methods have also been developed to quantify red and green snow algae using subtle shifts in spectral properties (Khan et al., 2020). In addition, hyperspectral reflectance contains information about the magnitude of scattering related to particle concentration and fluorescence of different types of pigments, such as chlorophyll a (Behrenfeld et al., 2009), among others (Dierssen et al., 2015). Underwater hyperspectral imaging has also been used to assess benthic flora and fauna on the seafloor using an autonomous vehicle with artificial lights (Dumke et al., 2018). Diverse classification techniques for hyperspectral imagery range from neural networks (Chirayath and Li, 2019) to semi-analytical inversion models. Miniaturized hyperspectral imaging spectrometers have also been successfully developed to assess the broad range of spectral requirements for remote sensing of both snow/ice and water (Bender et al., 2018).
Active Optical Remote Sensing (Independent of Solar Illumination)
Active sensors that produce and sense their own stream of light, such as radar/lidar (Radio and Light Detection and Ranging) are generally flown on aircraft, although several space-based lidars have also been launched. Lidar uses the round-trip travel time of a pulse of laser light to estimate distance to the seafloor (Dierssen and Theberge, 2012) or to particle fields. Aircraft-mounted systems pulse a narrow, high-frequency laser beam toward Earth and are capable of recording elevation measurements at rates of hundreds to thousands of pulses per second. Because they do not rely upon sunlight, lidars can be operated at night. These instruments have long been successfully used to assess the vertical structures of aerosols and thin clouds (Winker et al., 2003). Space-based green lidars are commonly used to assess the temporal and spatial characteristics of ice sheet elevation changes. The most advanced of these at present is the Advanced Topographic Laser Altimeter System (ATLAS), a six-beam, photon-counting lidar operating at 10 kHz that provides high spatial resolution measurements of altimetry with applications spanning sea ice thickness, vegetation canopy changes, and shallow water bathymetry (Abdalati et al., 2010; Gleason et al., 2021).
Lidars can be used to measure light backscattered off ocean particles (Behrenfeld et al., 2013) as well as fluorescence from chlorophyll and colored dissolved organic matter (CDOM; Hoge et al., 2005). Although elastic backscatter lidar is effective at retrieving vertical profiles of particle concentrations, it does not provide information about the composition of particles (mineral or organic). Applications of airborne lidar that also involve the use of fluorescence techniques with chlorophyll and CDOM provide more diagnostic information about particle content (Hoge et al., 2005). A significant leap in retrieval accuracy and particle information is also achieved with a high-spectral-resolution lidar (HSRL), which can provide an estimate of spectral light attenuation in the water column. Spectral light attenuation has long been used in oceanographic studies to estimate absorption properties of pigments and other organic matter in ocean waters (Smith and Baker, 1978). New research in the use of subsea lidar systems from ships that couples backscattering measurements with linear depolarization also has relevance for detecting the vertical distribution and optical properties of suspended ocean particles, including optical measurements related to absorption and index of refraction of particles (Zimmerman et al., 2013).
Active remote-sensing technologies such as radar and lidar are largely independent of ambient illumination conditions, provided there is sufficient transmitter irradiance over background, and advantageously contend with attenuation along the optical path by exploiting phase information using heterodyne receivers. Thus, hardware requirements for receiver sensitivity, aperture, and SNR can effectively be relaxed given increased transmitter power (up to MW of power in the case of radar). Recent lidar advances also allow use of multiple wavelengths of laser diodes simultaneously in green and two infrared bands to achieve a “color” point cloud (Briese et al., 2012, 2013).
MiDAR, a recently patented NASA invention of the year in 2019, is an active multispectral remote-sensing and optical communications instrument technology that is opening new frontiers in marine ecosystem imaging across the ultraviolet to near infrared spectrum. MiDAR can perform rapid underwater multi/hyperspectral imaging and operate in extreme and light-limited environments (Figure 3; Chirayath, 2018a,b). MiDAR is also designed with fluid lensing compatibility that will help to extend the depth range of the passive NASA FluidCam instrument intended for use in subaquatic remote-sensing applications in entirely light-limited environments, such as those on ocean worlds in the outer solar system (Figure 4; Chirayath and Li, 2019).
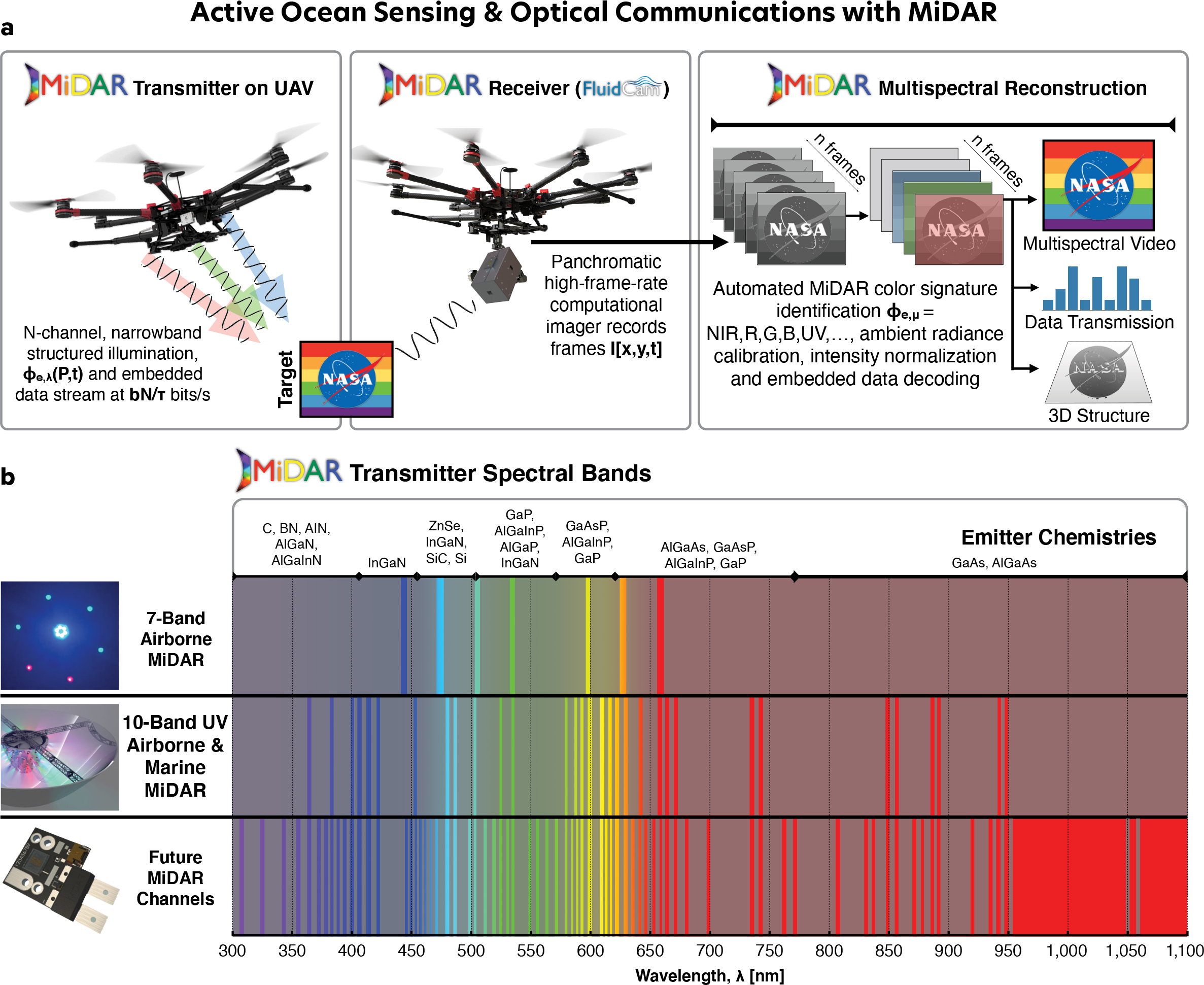
FIGURE 3. (a) NASA’s Multispectral, imaging, Detection, and Active Reflectance (MiDAR) instrument uses multiple narrowband optical emitters to illuminate a target with structured light (MiDAR transmitter). The reflected light is captured by a telescope and a panchromatic focal plane array (MiDAR receiver). Using a heterogeneous computing architecture, MiDAR creates hyperspectral images at video frame rates and decodes embedded optical communications in real time. The structured illumination pattern generated by the MiDAR transmitter permits simultaneous optical communication and calibrated measurement of a target’s reflectance at multiple wavelengths, independent of ambient illumination conditions, that may be particularly applicable to future ocean worlds robotic explorers. MiDAR can be operated in a bistatic or monostatic configuration on aircraft, spacecraft, and underwater and surface vessels. (b) Multiple MiDAR transmitters, both developed and presently under development, span the ultraviolet to near infrared optical spectrum for oceanographic applications on Earth. Emitter chemistries have been identified for each spectral channel that allow for high luminous efficiency exceeding that of lidar. Adapted with permission from Chirayath and Li (2019). > High res figure
|
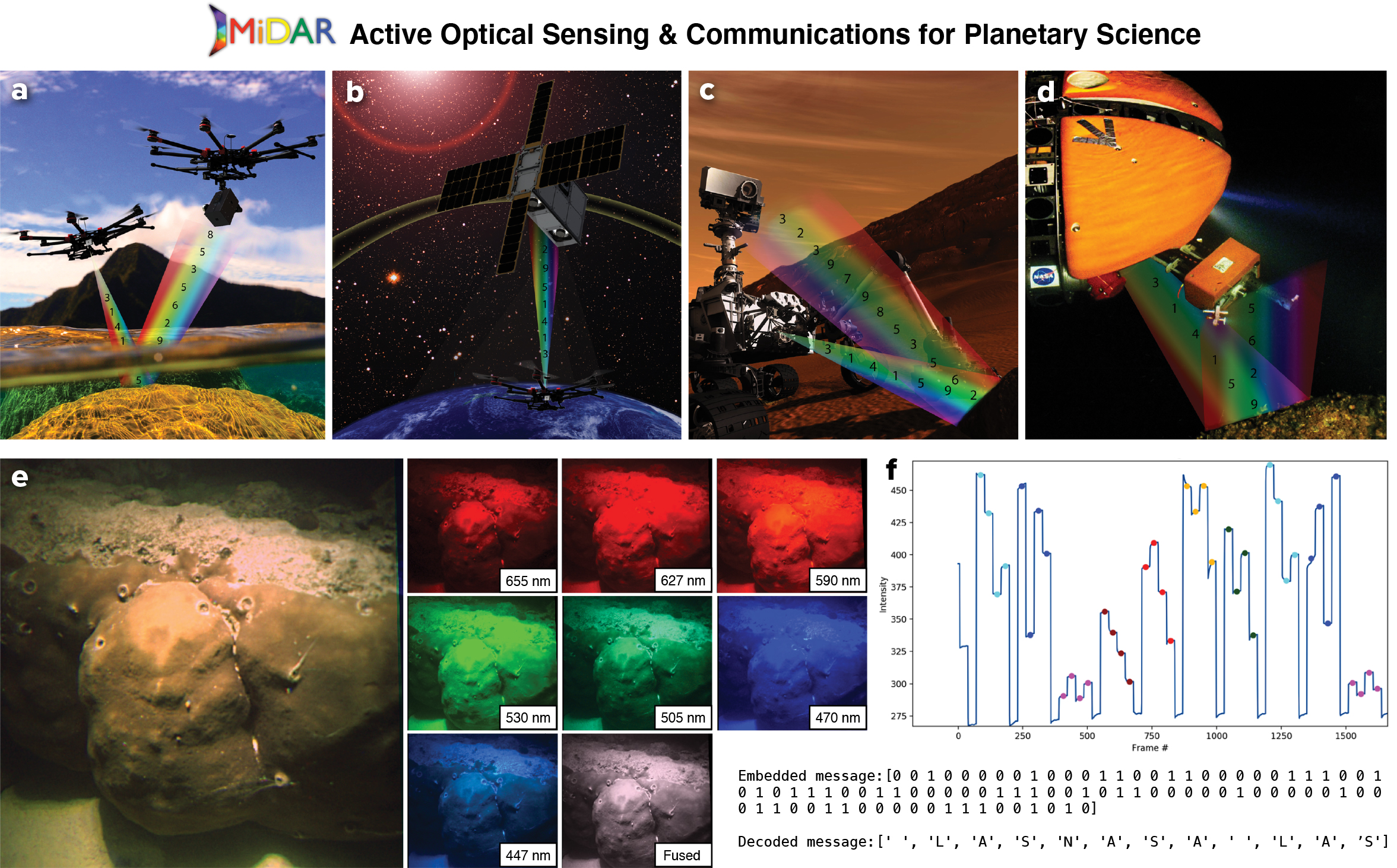
FIGURE 4. MiDAR Earth and planetary science applications. (a) Airborne MiDAR depicted operating over a coral reef. (b) MiDAR operating as high-bandwidth communications link to satellite system from airborne or underwater transmitter. (c) MiDAR integrated onto Mars rover for hyperspectral and ultraviolet sensing of facies. (d) MiDAR integrated onto a deep-sea robot for active multispectral benthic remote sensing and optical communications in light-limited environment. Background image credit: NASA (e) MiDAR field results from 2018 Guam campaign demonstrating active multispectral imaging and optical communications. Here, a seven-band MiDAR is operating in a bistatic configuration with a MiDAR transmitter above the surface and an underwater MiDAR receiver. MiDAR resolves a Porites coral and simultaneously performs simplex optical communication through the air-water interface (f). Embedded MiDAR data transmission in coral image encodes a hidden message recovered in real time. Adapted with permission from Chirayath and Li (2019). > High res figure
|
Synthetic aperture radar (SAR) is an active instrument technique in which a series of radar signals are transmitted from an observing platform (spacecraft, airplane). The signals bounce off the surface and then are received by an antenna to build an image that is viewed at radar wavelengths. The returned signal is referred to as backscatter, and a SAR image displays various amounts of backscatter. The amount of radar backscatter is sensitive to the grain-size roughness of materials at the scale of the radar wavelength, angular faces that reflect radar energy back (or away) to the receiver, volume scattering, dielectric constant of the material, and the presence of dielectric constant changes (layering). Due to its dependence on roughness and physical parameters, radar backscatter provides more complementary information than regular visible or infrared spectroscopy. Radar wavelengths are longer than visible and infrared wavelengths (radar is usually on the order of centimeters to tens of centimeters), and thus interrogate deeper into the surface than visible spectroscopy, on the order of tens of centimeters, with the exception of liquid water (see Ager, 2013, for an in-depth description of this technique).
Planetary SAR has been useful on worlds where there are thick or obscuring atmospheres that render shorter-wavelength imaging impractical, such as Venus and Titan (Elachi et al., 2004, 2005, 2006). The Cassini spacecraft used SAR to extensively examine the surface of Saturn’s haze-shrouded moon Titan (Lopes et al., 2019), imaging such surface morphologies as dunes, dissected plateaus, craters, lakes, and channels at high resolution. The SAR data was used to define Titan’s terrain unit classification system and enabled construction of a global geological map (Malaska et al., 2016).
Radar altimeters have been hugely successful at observing sea surface height on Earth through missions such as TOPEX/Poseidon, Envisat, Jason-1, and Ocean Surface Topography Mission/Jason-2. For non-ice-covered ocean worlds, such measurements of sea surface height would enable discoveries about geostrophic, wind, and thermally driven dynamics. Other instrument techniques include microwave emissivity, a passive technique for detecting microwave (radar) energy using an antenna. It uses natural radio emission to determine the brightness of a terrain and can be performed during SAR acquisition when signals are not being actively received or at larger distances where SAR is not practical. When coupled with an understanding of the physical temperatures, microwave emissivity can provide information on grain size, volume scattering, and material properties (Le Gall et al., 2016). For example, many of Titan’s terrains exhibited a characteristic microwave emissivity that allowed differentiation between terrain units and between organic terrains and icy terrains (Malaska et al., 2016, 2020b).
Ice- and Ground-Penetrating Radars
Ice-penetrating radar sounding is a primary geophysical method for subsurface observation of terrestrial ice sheets and ice shelves (Schroeder et al., 2020). It has also played a leading role in the subsurface exploration of Martian and cometary cryospheres and is included in the payloads of planned missions to the ocean worlds of Ganymede (Bruzzone et al., 2011) and Europa (Blankenship and Young, 2018). Recent advances in radar sounder data analysis have enabled improved characterization of subglacial (Jordan et al., 2018) and englacial (Chu et al., 2018) water systems as well as ice shelves (Grima et al., 2019) and grounding zones (Christianson et al., 2016); these can be adapted to the exploration of ocean worlds’ ice shells for detection and characterization of liquid subsurface water (Michaelides and Schroeder, 2019). Similarly, new approaches to constraining the attenuation, temperature structure, and advection/melt rates of terrestrial ice sheets (Winebrenner et al., 2019) can be applied to constraining the thermophysical structure of ocean world ice shells (Kalousová et al., 2017). Finally, stationary active and/or passive radar sounders offer the ability to create time-series observations of subsurface conditions from an ocean world lander. Adapting and expanding this rich array of terrestrial radio glaciological techniques to the exploration of ocean worlds promises to dramatically increase the subsurface geophysical capabilities of both earthbound and space-oriented scientific communities.
Plume Sampling
For sampling plumes at actively venting worlds, such as Enceladus or possibly Europa, an orbital-speed plume fly-through mission is a potential option. Mass spectrometry using impact-induced ionization is an effective means of detecting inorganic and organic molecules entrained within ice grains, though care must be taken when selecting sampling speeds. Cassini spacecraft instruments sampled the Enceladus plume gases and grains at hypervelocity (7–17 km s–1) during multiple flyby encounters, and detected H2, NH3, CH4, Ar, silica nanograins, salts, and simple and complex organic molecules (Sekine et al., 2015). Recent focus has been on developing advanced instrumentation to determine biotic and abiotic distributions (Klenner et al., 2020b) and on predicting optimal encounter velocities, which are thought to be 3–6 km s–1 for amino and fatty acids (Klenner et al., 2020a). The SUrface Dust Analyzer (SUDA) aboard the Europa Clipper spacecraft will analyze dust sputtered from the moon’s surface. A return mission to Enceladus, to analyze the plume with particular emphasis on biosignature detection and quantification, and a new mission to Triton, to determine if it hosts a subsurface ocean and to characterize its plumes, are both in concept stages.
Plume fly-through sampling and analysis tools are under development and testing in terrestrial settings. For example, LACROSS (Life Analysis, Capture, and Retention on an Orbiting Saturn Spacecraft) is a Raman instrument concept designed to minimize sample alteration and maximize signal over noise for analysis of plume ices in ocean worlds (Sobron et al., 2018). Plates have been designed to capture plume material (New et al., 2020) and shown to be capable of collecting particles containing organics at velocities of up to 1–2 km s–1. Organic molecule capture efficiencies at these velocities range from 10%–50% for more than a quarter of the particle impacts on the plate. A funnel collector could be positioned on an external surface of the spacecraft to collect plume material at Enceladus or other planetary bodies that exude icy material (MacKenzie et al., 2020). The material would flow to a collection system at the base of the funnel for capture and transfer to internal instrumentation that would prepare and analyze samples. One such funnel, the EFun collector (Adams et al., 2018), is in development for capturing ice particles.
In Situ Technologies
In situ technologies for ocean worlds span systems and instruments that operate above, on, under, or within ice, slush, and water. Terrestrial oceanographic measurements and sensing technologies that determine biological, chemical, and physical properties and that are relevant to understanding ocean worlds are summarized here.
Robotic Platforms, Access, and Sampling Technologies
Significant progress has been made over the past decade in characterizing the seafloor, the ocean surface, and the water column over large geographic areas using underwater remotely operated or autonomous underwater vehicles (ROVs and AUVs; Roberts et al., 2010), unmanned surface vehicles (USVs; Mordy et al., 2017), profiling floats (Roemmich and Gilson, 2009), and unmanned aerial vehicles (UAVs). Three-dimensional photogrammetry, active acoustical methods, and in situ water column measurements have been used with remarkable effectiveness on such platforms to narrow the gap in observational capacity between terrestrial and aquatic systems, revealing mesophotic and deep-sea habitats with unexpected biodiversity and ecological complexity (Pizarro et al., 2004).
Sub-ice-shelf instrumentation and ROVs have been deployed through hundreds of meters of ice using hot-water and electro-mechanical drilling methods. For example, the IceFin vehicle was deployed, most recently, in 2019–2020 beneath approximately 600 m of ice in Antarctica’s Thwaites Glacier and the Ross Ice Shelf (Meister et al., 2020) using hot-water drilling technology similar to that described by Makinson and Anker (2014). Distributed temperature sensing fiber-optic cables were deployed through 193 m of ice in and below the McMurdo Ice Shelf using an electro-mechanical drilling method (Tyler et al., 2013). However, logistical costs for these methods increase rapidly with increasing ice thickness and decreasing ice temperature. This has spurred development of ice melt probe technology in which modern materials and methods are used to make melt probes far more reliable than the first such probes (which were developed in the 1960s and 1970s; Winebrenner et al., 2016). Melt-probe technology is thus now among the candidates for outer solar system flight hardware.
A number of existing and developing subsurface access technologies are available to serve both terrestrial and ocean worlds missions. Underwater sampling tools include flow-through systems developed for terrestrial oceans, including the McLane Remote Access Sampler (RAS), AquaLAB (Dodd et al., 2006), and the Monterey Bay Aquarium Research Institute’s 3G-ESP (third generation Environmental Sample Processor). Drills used to explore shallow subsurface environments have been flown to Mars, for example, the Phoenix and InSight landers, and the TRIDENT drill is a 1 m class shallow drill in development for lunar access. For deeper access, drills currently in development for this decade’s moon and Mars missions aim to access 10 m and deeper and can inform ocean worlds technology developments (Dachwald et al., 2020). Other technologies under development for ocean world access through ice, in particular via the NASA Scientific Exploration Subsurface Access Mechanism for Europa (SESAME), include the VALKYRIE (Very-deep Autonomous Laser-powered Kilowatt-class Yo-yoing Robotic Ice Explorer) mechanical and hybrid thermo-mechanical drills (Stone et al., 2018); SLUSH (Search for Life Using Submersible Heated Drill; Zacny et al., 2018); an ice melt probe (Winebrenner et al., 2016); and a cryobot combining these methods (Cwik et al., 2019).
Ice and soil sample collection technologies have been developed for Mars missions and for Europa, mostly consisting of surface disruption/cutting tools and scoops (Scoops: Phoenix mission, Honeybee Robotics; Europa Lander scoop, Jet Propulsion Laboratory. Rasps: Badescu et al., 2019, Jet Propulsion Laboratory. Pneumatic transfer: Kris Zacny, Honeybee Robotics, COLDTech. Plume fly-through collection devices, including funnels and impact plates, for example, COLDTech, Elena Adams, Johns Hopkins University Applied Physics Laboratory).
Milli- and micro-fluidic systems are in development to bring in and process ice to liquid samples. Grants under the NASA Instrument Concepts for Europa Exploration (ICEE) 2 program are particularly focused on sample processing and analyses for a Europa Lander type in situ mission to search for biosignatures. Other instrument development grants that also focus on ocean world in situ technologies include Planetary Instrument Concepts for the Advancement of Solar System Observations (PICASSO) and Maturation of Instruments for Solar System Exploration (MatISSE), and COLDTech.
Biological and Geochemical Sensing Technologies
Oceanographic sampling technologies include automated water samplers for carbonate chemistry (Enochs et al., 2020), for oceanographic time series (van der Merwe et al., 2019), for deep-sea microbial sampling (Peoples et al., 2019), and for automated eDNA sampling (Nguyen et al., 2019; Yamahara et al., 2019). These technologies also include biodiversity sampling systems that employ settlement plates; an example is autonomous reef monitoring structures (ARMS), which can reveal marine biodiversity, cryptic biodiversity, community structure, and microbial community diversity via high-throughput DNA sequencing methods (Pearman et al., 2019).
Autonomous sensor technology is well established for assessing water quality across Earth’s aquatic ecosystems (IOCCG, 2018), including measurements of the optical properties of different dissolved and particulate matter (Werdell et al., 2018) that are used in conjunction with radiative transfer models for interpreting the mixtures of these materials (Twardowski and Tonizzo, 2018). The color of light reflected from or within a water body is related to the absorption and scattering properties of different types of dissolved and particulate matter, as well as inelastic processes like Raman scattering and fluorescence. Distinct spectral shapes created by absorption of light by dissolved humic and nonhumic substances, such as pigment-like components, amino acid or protein-like components, and small colloids called CDOM or gelbstoff, can be measured optically both from space and in the field. Measurements of particulate absorption can be used to assess living phytoplankton of a wide range of sizes and shapes, as well as larger detritus from living cells, suspended minerals, and sediment. In addition, in situ laser diffraction measurements can assist in determining the size distribution of sediment grains resuspended in plumes and of algae in blooms in water (Buonassissi and Dierssen, 2010). When seeking life on other ocean worlds, such optical measurements can provide direct evidence of the types of organic and inorganic matter found within an ocean world. Also applicable to ocean worlds are optical oxygen optodes (Bittig et al., 2018), spectrophotometric nutrient sensors (Johnson and Coletti, 2002), and pH and pCO2 sensors (though novel ocean world chemistries may motivate further development here).
To make in situ detections of biosignatures at planetary environments, several instruments are in development, including the Enceladus Organic Analyzer for detecting amino acids (Mathies et al., 2017), the Search for Extra-Terrestrial Genomes (SETG) instrument for nucleic acid detections (Bhattaru et al., 2019), the Europan Molecular Indicators of Life Investigation (EMILI) for detecting molecular biosignatures, and others. Novel, small, low-mass and low-power nanopore devices have been developed commercially that can detect, and in some cases characterize, long chain polymers (LCPs), including DNA, RNA, and proteins as they pass through the pore, for example, the MinION by Oxford Nanopore Technologies Ltd. Although this technology has been demonstrated on the International Space Station (Castro-Wallace et al., 2017), we note that it uses biologic protein pores that are susceptible to degradation over long space missions and in high radiation environments. Synthetic nanopore devices are being developed (Xue et al., 2020) that would be more robust to these conditions because they can form pores of various sizes to assist with detections; however, their usefulness is challenged by increased flow-through speeds that reduce sensing resolution.
In situ remote instrumentation is available that allows emplacement and monitoring while being embedded in ice. On Earth, these instruments can be used to measure the electrochemical parameters of meltwater, reveal the residence time and likely chemical weathering history of percolating meltwater, and determine links between sub-ice pressure and ice dynamics.
Raman spectroscopy is also an excellent tool for analyzing biosignatures. Raman can be used to detect and (semi)quantitate minerals and organic compounds, and it is particularly sensitive to biominerals such as silica, carbonates, sulfates, magnetite, and metal oxides. Raman can also be used to detect salts (e.g., chlorides, sulfates), silicates, metals, metal (oxy)hydroxides, volatiles (e.g., CH4; COx), and radiation products (e.g., H2O2 O2) (Nakamoto, 2008). This ability for broad chemical characterization greatly increases confidence in interpretation of the origin of minerals and organics. Many of these compounds help constrain habitability parameters, such as the extent and style of water-rock interactions in the ocean; ocean pH, salinity, and redox state; the presence of bioessential elements (carbon, hydrogen, nitrogen, oxygen, phosphorus, and sulfur); and the availability of redox couples that life can use as an energy source. The Raman instrument SHERLOC (Scanning Habitable Environments with Raman & Luminescence for Organics & Chemicals) flew along with SuperCam on NASA’s Mars 2020 rover, and the Raman Laser Spectrometer (RLS) is to be included in the European Space Agency’s ExoMars rover. Raman instruments are also being considered for flight in the Europa Lander Mission. Instruments currently in development, such as the in situ Spectroscopic Europa Explorer (iSEE), will further increase Raman sensitivity for biosignature detection purposes (Sobron et al., 2018).
Fluorescence and Raman spectroscopy can reveal information about the electronic and rovibrational states of a molecule. Molecules that fluoresce include aromatic amino acids such as tryptophan, tyrosine, and phenylalanine, as well as aromatic molecules such as benzene, naphthalene, and other polyaromatic hydrocarbon species (Bhartia et al., 2008). Fluorescence has the advantage of being very sensitive, with low limits of detection and quantitation. Raman spectroscopy determines rovibrational states based on functional group atom-atom vibrations, rotations, and stretching. In general, Raman is less sensitive when compared with fluorescence spectroscopy, but it can be enhanced by using excitation wavelengths that are preferentially absorbed by the molecule in a technique called fluorescence-enhanced Raman (Sapers et al., 2019). The use of Deep UV excitation allows absorption of ultraviolet photons by aromatic molecules, which provides both fluorescence and fluorescence-enhanced Raman signals (Bhartia et al., 2008).
The current state-of-the-art instrument for planetary applications is the Mars Perseverance SHERLOC instrument, currently operating on Mars (Beegle et al., 2015). SHERLOC can map a 7 cm × 7 cm surface with a 50-micron spot laser and acquire both fluorescence and Raman spectra. A companion instrument (WATSON, Wide Angle Topographic Sensor for Operations and eNgineering), which was shown to be effective when attached to the planetary deep drill in the Greenland Ice Sheet for down-borehole applications in ice and rock, uses a similar strategy, but the optical path and component have been reconfigured to fit into a tube (Malaska et al., 2020a). While the SHERLOC instrument is designed for study of surface reflectance, the transparent nature of ice and evaporite deposits (gypsum, halite) allows WATSON to penetrate the interior of the matrix (Eshelman et al., 2019).
Communications
Relaying data from underwater instruments to and through the surface at bandwidths common to airborne and spaceborne platforms has remained a significant obstacle to sustained deep-sea mapping on Earth. Robotic explorers on ocean worlds will need to communicate with one another and/or an orbital platform from challenging environments on a dynamic icy surface, under ice, and submerged in fluid or slush.
Several technologies are being proven on Earth with future applicability to ocean worlds. NASA’s MiDAR has demonstrated simultaneous active multispectral imaging and optical communications through a dynamic air-water interface (Figure 4; McGillivary et al., 2018; Chirayath and Li, 2019). Future planned experiments with MiDAR include deep-sea benthic and hydrothermal vent mapping as well as optical communications through turbid environments. Recent developments have allowed wireless radio frequency sensor platforms to return data from the seabed to the ice surface. “Cryoegg” is a spherical, very high frequency subglacial probe that has been proven through 1.4 km of Greenland ice (Prior-Jones et al., 2020). Its 15 cm diameter allows deployment via standard ice core boreholes, followed by free-roaming in subglacial meltwater channels. It has been tested in moulins (Bagshaw et al., 2014) and deep boreholes (Prior-Jones et al., 2020) in Greenland, with data returned in real time from a depth of 1.4 km. The current Cryoegg instrument measures simple electrochemical parameters (temperature, pressure, electrical conductivity), but the sensor suite is interchangeable and more complex biogeochemical sensors may be incorporated into the platform (e.g., pH or dissolved oxygen).
Two other wireless systems have been tested in subglacial environments. The WiSe (Wireless Subglacial Sensing) system (Smeets et al., 2012) was capable of returning a signal through 2.5 km of ice in Greenland. The Glacsweb system (Martinez et al., 2004) was also borehole-deployable, with a lozenge-shaped probe designed to lodge in subglacial till. Planetary applications of these systems would allow emplacement and sensing in the deep ice crusts of ocean worlds for long duration monitoring of changing chemical conditions, for example, during crustal flexure due to tidal cycle.
Concluding Remarks
Earthbound oceanic explorers continue to motivate and invent exciting new technologies that uncover new species, mechanisms, and environments unknown to science. These efforts are bearing fruit at a crucial inflection point in our species’ time on Earth. New technologies are revealing, more than ever, just how dependent our species’ survival is on a healthy ocean (Purkis and Chirayath, 2022). Look no further than a sea squirt to find the origin of one the most potent COVID-19 medications derived to date. Yet, these new tools are also uncovering just how much the ocean is changing as a consequence of our actions. Simultaneously, ocean worlds across our solar system are being revealed in ever more detail, offering new analogs for comparison to Earth’s ocean and igniting the effort to discover extraoceanic life and novel oceanic biogeochemical planetary processes. Studying these ocean worlds will provide information that is unique and crucial to preserving life on our own world.
Here, we aimed to survey some of the state-of-the-art technologies being used across oceanography and planetary science to reveal the biogeochemical properties of oceans. These and other technical developments portend an exciting future ahead for better understanding the ocean we depend on, and crucially enabling the robotic exploration of other ocean worlds in the upcoming decades. Exploration of the rocky planetoids of our solar system informed terrestrial geology and uncovered the minimal conditions for terrestrial life. Having planetary analogs for the ocean on our celestial doorstep is fortunate indeed, especially if the study of these worlds can better inform the uniqueness and fragility of our blue planet.
Acknowledgments
This work was made possible with support from NASA’s Ocean Biology and Biogeochemistry Program, Biodiversity and Ecological Forecasting Program, Astrobiology Program, ICEE-2 grant, and internal University of Miami and APL funding.