Introduction
A diverse array of planetary bodies in the outer solar system that are now thought to harbor deep liquid water oceans beneath their thick ice shells are termed ocean worlds. All identified since the turn of the millennium, they include five moons of the “gas giants” Jupiter (Europa, Ganymede, Callisto) and Saturn (Enceladus, Titan) for which direct evidence of global-scale subsurface oceans has already been obtained (Nimmo and Papallardo, 2016). There are also a larger number of “candidate” ocean worlds where liquid water may exist, based on the sparse data collected to date and awaiting future confirmation (Hendrix et al., 2019). One area of particular interest is a subset of ocean worlds already confirmed to host large-volume oceans and where water-rock reactions could render them habitable (Hand et al., 2020; Howell and Papallardo, 2021).
NASA’s Ocean Sciences Across the Solar System initiative directly responds to these new discoveries and considers how ocean and planetary researchers can best collaborate to accelerate our understanding of how ocean worlds function, including on our home planet, Earth. Topics explored elsewhere in this special issue include study of life in Earth’s hydrobiosphere (Hoehler et al., 2022), what constitutes habitability in an ocean world environment (Glass et al., 2022), the technologies required to advance ocean world exploration (Chirayath et al., 2022), and the selection of suitable analog environments on Earth relevant to the predicted physics, chemistry, and potential biology of other ocean worlds (Arrigo, 2022). Here, we consider how our understanding of processes in Earth’s oceanic environments can be coupled with the sparse data available from space to make meaningful theoretical predictions about other ocean worlds. Specifically, we advocate for an approach in which predictive modeling is constrained by laboratory experimentation as well as direct observations of Earth’s ocean, which will help to guide the development and application of innovative technologies that will be required for future space missions. Our approach is integrative, spanning multiple interrelated subdisciplines that consider processes from the subseafloor to the ocean-ice interface. In brief, we advocate for applying ocean systems science to the future investigation of ocean worlds beyond Earth.
A major motivation for this work is to consider whether newly discovered ocean worlds in our outer solar system can host life and, if so, how we might be able to detect it. Put simply, we seek to address the question: On which ocean world(s), and with what measurements, would we have the greatest opportunity to acquire definitive evidence for the presence of life beyond Earth? This search for chemosynthetic life on ocean worlds, as in Earth’s ocean, is neither a purely biological nor a multidisciplinary effort, but truly interdisciplinary. As we pursue this search, it is useful to define two key parameters—biological potential and biosignature potential:
- Biological potential: the abundance and productivity of life that an ocean world system has the capacity to support. Geophysical and geochemical processes determine whether the right conditions can arise on a given planet or moon to render it habitable and to constrain how much chemical energy is bioavailable for life (i.e., what is the nature of the chemical species present and at what rate are they available).
- Biosignature potential: the nature and abundance of evidence for life that an ocean world has the capacity to manifest. Transport by physical oceanographic processes coupled with (bio)geochemical cycling within the oceans of any ocean world will dictate where any (potentially diagnostic) tracers of a planetary body’s habitability and/or any life it may host are dispersed. These factors also help to determine whether these signatures of habitability and/or life might be expressed outward as far as an ocean world’s icy exterior, or whether they would be trapped within.
Figure 1 illustrates the relationship between biological potential and biosignature potential in schematic form. Biosignature potential is of particular importance in planning future space missions because it helps define what instruments any mission to an ocean world should carry and where those measurements should be made on the target ocean world. However, biological potential is at least as important because it provides insights into the likelihood that any given ocean world might host life, regardless of how challenging it might be for a mission to detect its signature.
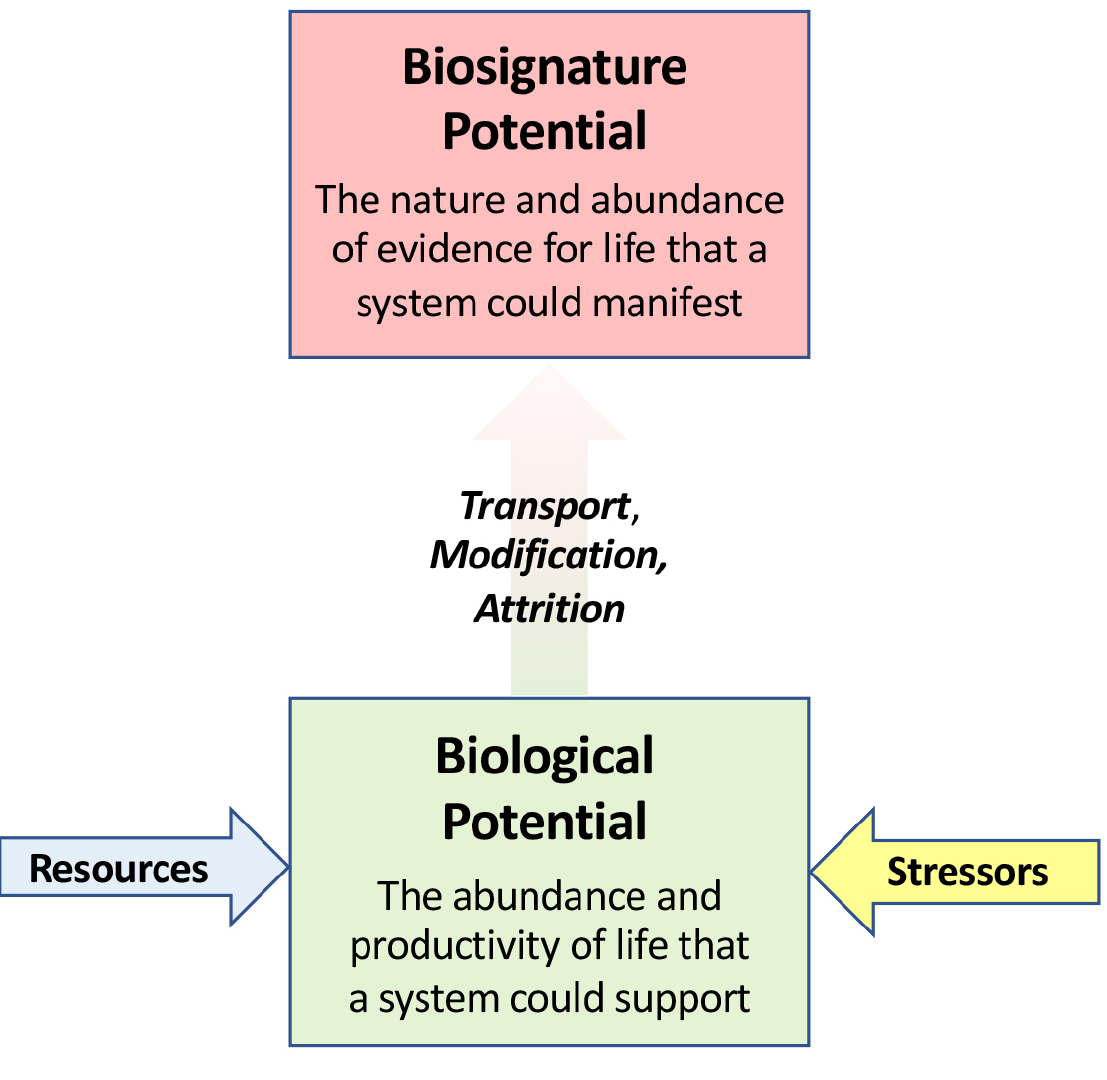
FIGURE 1. Schematic representation of what constitutes the biological potential and the biosignature potential of any given ocean world and the interrelationship of the two. See text for expanded definitions of biological potential and biosignature potential. > High res figure
|
When contemplating the possibility of life beyond our planet, it is important to remember that not all life on Earth relies on photosynthesis fueled by energy from the sun. Part of our biosphere is driven by chemosynthesis involving microbes that harness the energy of simple chemical species produced via fluid-rock interactions—for example, at submarine hot springs on Earth’s deep ocean floor. Therefore, wherever a rocky seafloor and a saltwater ocean interact beyond Earth, it is conceivable that habitable conditions—and perhaps life—might exist. Not all ocean worlds have liquid water in contact with a rocky seafloor—some have water sandwiched between two different forms of ice (one low pressure and one high pressure; Nimmo and Papallardo, 2016). But two already confirmed ocean worlds, Jupiter’s moon Europa and Saturn’s moon Enceladus, exhibit these foundational conditions for habitability (Hand et al., 2020). Numerous additional candidate ocean worlds have also been identified in our outer solar system (Hendrix et al., 2019), and a high proportion of extrasolar planets are now also predicted to be ocean worlds (Quick et al., 2020). Recognizing that chemosynthesis has long been present as a life-sustaining process on Earth, and that it supports expansive biological communities in Earth’s ocean, our concept of the habitability and, hence, biological potential of the universe has expanded vastly in recent decades, beyond just the canonical “goldilocks” zone where proximity to a parent star allows liquid water to exist at the surface of a planet.
Applying an ocean systems science approach can help us address these fascinating problems. In the remainder of this article, we describe approaches to investigating the concepts of biological potential and biosignature potential. Figure 2 shows the physical processes that we discuss extending from the interior of a planetary body outward. We focus specifically upon conditions that extend from the planetary surface, through the ocean, and into the rocky interior. Given the expertise of this author group, the cryosphere is not considered in detail, but should be integrated more holistically with ocean-based studies in the future.
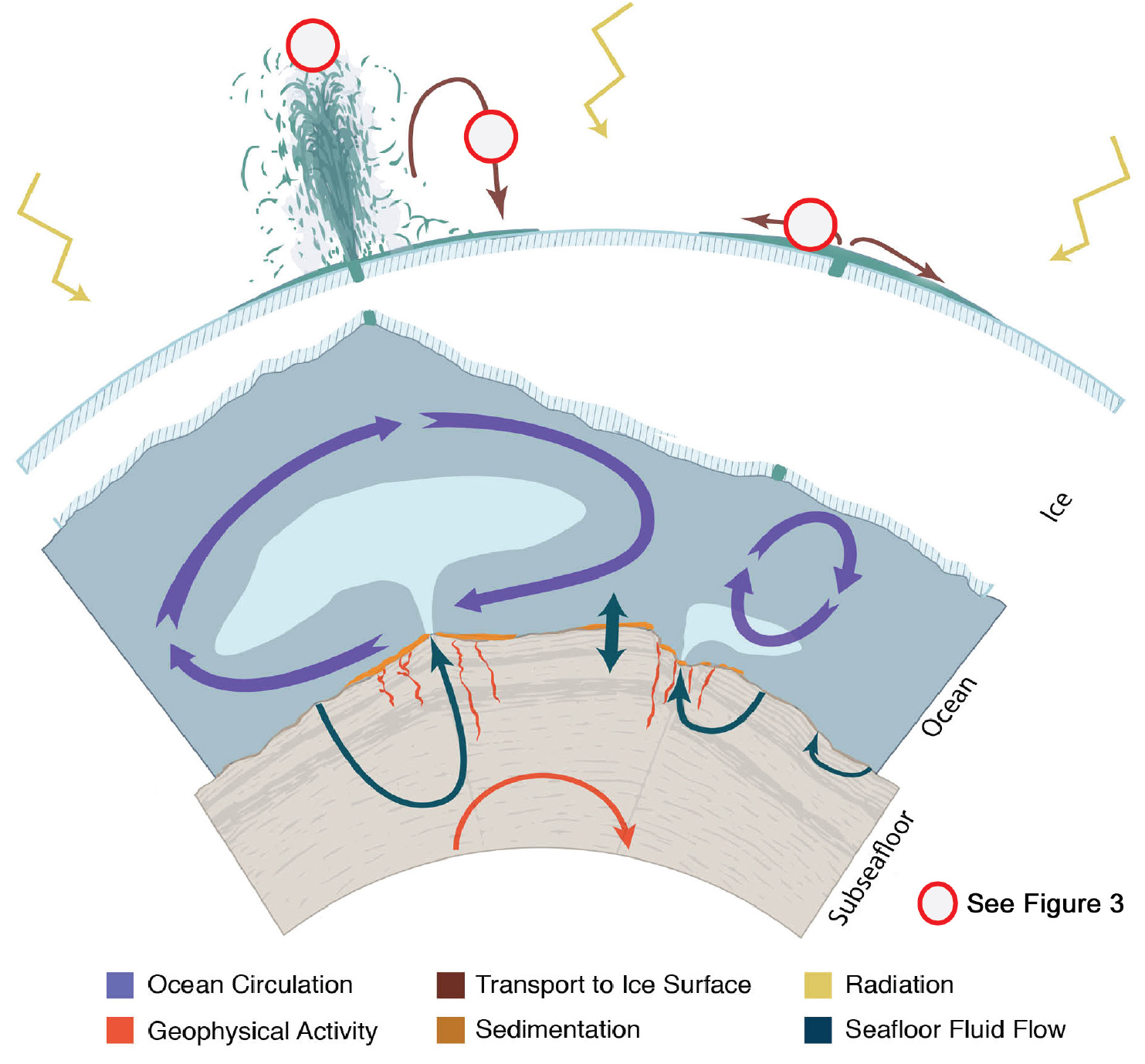
FIGURE 2. Artist’s impression of the physical processes pertinent to an ocean system science approach to investigating processes beneath the ice shell of an active ocean world. Red circles with white centers indicate candidate locations on other ocean worlds where marine particles derived from the subsurface ocean might be expressed and preserved. > High res figure
|
Biological Potential
For an ocean world to host life, it must have chemical energy sources that can act as electron donors and acceptors. For the geological conditions to arise that provide this chemical energy, however, there must be ongoing or recent release of thermal energy. One source of such heating might come from geophysical processes within the planet’s interior, for example, from the latent heat of cooling of magmatic fluids within the body’s rocky interior, from exothermic reactions, or from decay of K, U, and Th and other shorter-lived radiogenic isotopes trapped within the body’s interior at the time of formation (e.g., Humphris, 1995; Kelley et al., 2002; Morgan et al., 2016). Another important source of heating identified for both Enceladus and Europa is tidal/frictional heating related to the orbital interactions of each with their sibling moons and host planets (Travis et al., 2012; Spencer and Nimmo, 2013). Those areas of ocean world research fall more centrally within the domain of planetary scientists than of oceanographers (e.g., see Nimmo and Pappalardo, 2016). Earth’s ocean scientists, however, have a wealth of expertise in understanding how geophysical processes within a planet’s interior can influence both heat flux and the possibility of lateral variability in the character of an ocean world’s seafloor. The material properties of this rocky component of an ocean world will also be important in controlling the hydrogeology of any fluids that circulate through, extract heat from, and react chemically within the subseafloor environment. Importantly, even on a planet that has become relatively geologically inactive, residual topography in a submarine environment can impart lateral differences in pressure or heat flow at the seafloor that could be sufficient to support subseafloor fluid circulation. Conversely, sediment deposition at the seafloor—whether from mechanical degradation of topography, (bio)geochemical precipitation from the ocean, or particles from exogenic sources transmitted through ice into the ocean—might have a counterbalancing impact, inhibiting convection wherever ocean currents might distribute them across the seafloor.
The physical regime of an ocean world will determine the reaction conditions at which ocean-derived fluids and the rocks that those fluids cycle through interact; pressure, temperature and flow rate are all important in dictating the products generated from any set of reactants. Of course, on other ocean worlds, we have less direct knowledge of what the interior rock or ocean compositions might be. On Earth, which has undergone internal differentiation, the dominant rock type at the seafloor is basaltic in composition, but submarine vent fluids also interact with more silicic calc-alkaline rocks and ultramafic rocks in a variety of geological settings (e.g., MacGeehan and MacLean, 1980; Hodgkinson et al., 2015). Mafic and ultramafic submarine hydrothermal systems may be the most likely to be encountered on other ocean worlds whether or not their rocky interiors have undergone any form of internal differentiation, assuming the starting material for that body’s rocky interior was similar to that of chondritic meteorites in bulk composition. Key variables that influence the composition of ocean fluids circulating through the rocky interior of any ocean world, and their potential to yield chemical energy, include the total salt content of the ocean, the dominant ionic species present, and the balance of chemically oxidized and reduced species present in the ocean. Combining the spectrum of possible reactant compositions with the pressure, temperature, and flow rate for subseafloor fluid flow allows a wide array of possible chemical reactions to be anticipated that can generate varying concentrations and fluxes of reaction products that can be released back to the overlying ocean.
On Earth, seafloor vent fluids that have circulated through the subseafloor can be compositionally distinct from the downwelling ocean water, leading to chemical disequilibria and a multitude of diverse reduction-oxidation reactions at and across the rocky interior/ocean boundary (Von Damm, 1995). This, in turn, can drive a variety of different chemical reactions to be exploited metabolically by microbes that form the base of chemosynthetically fueled food chains and underpin lush, oasis-like seafloor ecosystems (Dick et al., 2013). Theoretical modeling can be used to predict the cumulative outputs of such chemical reactions, together with their potential energy yield. Importantly, however, such predictions hinge upon what happens when chemical reactions proceed to chemical equilibrium. This process can be examined in the laboratory by identifying kinetic barriers that play fundamental roles in regulating fluid compositions. For example, under conditions where the energetics to support life are predicted to be particularly favorable, laboratory experiments to simulate the same reactions can be conducted both to test the model predictions and to investigate how kinetically controlled processes permit the formation of environments characterized by an abundance of potential chemical energy that can support life. Such studies can help us better imagine the bioenergetic opportunities and challenges that living organisms might face on another ocean world.
Of course, on other ocean worlds, the chemosynthetic processes that are most familiar on Earth need not arise at the ocean/rocky interior interface. In an ocean that is more chemically reducing than Earth’s, for example, but with a more oxidizing environment in the overlying ice shell, the entire ocean could be relatively reducing, and the release of chemical energy might be focused at the overlying ice-ocean interface. In the Arctic Ocean, similar life-sustaining processes have been observed at this interface (Arrigo et al., 2012; German et al., 2019). There, the flux of incident light penetrating the thin outer shell of sea ice is sufficient to drive the growth of photosynthetic algae attached to the underside of ice, and these algae in turn fuel their own local food chains. Beneath the thick ice shells of outer solar system ocean worlds, no such source of light for photosynthesis can be expected. But in the case of Europa, radiation from host planet Jupiter can lead to spallation and fragmentation of water molecules at the exterior of the ice shell. This process builds up oxygen molecules as some of the hydrogen escapes to space. Because the Europa ice shell is continuously being recycled (Katterhorn and Prockter, 2014), this oxygen may be transported to depth, where life could potentially exploit chemical energy generated as oxidized products from the ice shell are delivered to an underlying chemically reduced ocean (Russell et al., 2017).
Extending this discussion further, it is also possible that such interfaces on an ocean world could be completely decoupled from any liquid-solid interface. Examples of intra-water column redox interfaces that are both biogeochemically and microbially active within Earth’s ocean are well known, ranging from the permanently anoxic deep waters of the Black Sea (Murray et al., 1989) to the surface waters of hyper-saline brine basins in the Red Sea, the Eastern Mediterranean, and the Gulf of Mexico (Merlino et al., 2018). Moreover, vast tracts of oxygen deficient zones (ODZs) result from respiration beneath high-productivity surface waters within the mid-water column of the Arabian Sea and throughout much of the eastern Pacific Ocean from the northwest coast of the United States to the northern margins of Chile (Helly and Levin, 2004). It is important to note in this context that interior chemical gradients (redox or otherwise) are closely linked to the density stratification processes that drive ocean circulation. On Earth, the top-to-bottom density range is set by heat and freshwater fluxes at the sea surface and turbulent mixing throughout the water column, driven primarily by tides and winds, a process that replenishes the energy lost during the formation of deep and bottom waters (e.g., Munk and Wunsch, 1998). In the case of oceans covered by ice shells, the expected range of water densities is much reduced, and it is far from clear whether such oceans will be stably stratified in density.
What is critical, however, regardless of where the chemical energy might originate to promote the habitability of an ocean world, is the continuous formation of redox disequilibrium. Such disequilibria have already been shown to be present in at least one instance with the identification of both H2 and CO2 coexisting in plumes ejected from the oceans of Enceladus (Waite et al., 2017).
Biosignature Potential
The second key consideration when preparing to investigate ocean worlds—at least from an astrobiological perspective—is where any evidence of habitability (± any life that it supports) might be distributed and preserved in detectable form. In the case of an ocean world with habitable environments at the upper boundary of the ocean at its ice-ocean interface, the potential for detecting biosignatures may present less of a challenge. Jets of ocean-derived material ejected into space from the south pole of Enceladus have already been directly sampled by the Cassini Mission, and similar jets ejected from Europa have provisionally been detected using the Hubble telescope. These jets might tap directly into the uppermost ocean and, thus, include habitable/inhabited waters from the ice-ocean interface. But further complications might arise. For example, liquid water injected upward into the icy crust might not immediately reach the outer surface. Just like magmatic injection into Earth’s oceanic crust, ocean water (with or without biosignatures) might first pond in sills perched within the solid icy shell. It is important to consider what other important processes might then come into play. For example, could progressive freezing and brine exclusion lead to a concentration of key ingredients forming a microcosm/incubator of residual fluid—a cryospheric equivalent of Darwin’s warm little pond1 (Darwin, 1887)? Or, could the increase of salinity render that pocket of fluid sterile?
Whatever might occur within the ice shell of an ocean world, it is most likely that the source of chemical energy required to support life on that ocean world originates from seafloor-ocean interactions. To be detectable at the ocean world’s surface, within its cryosphere, or at the base of its ice shell (in the case of a future cryobot-enabled shell-penetrating space mission), any geochemical ingredients required to render it habitable, or any biologically derived products indicative of life, must be transported from the water-rock interface. On Earth, vertical transport of hydrothermal material released at the seafloor to the surface is accomplished primarily by the global overturning circulation, which is associated with timescales of years to centuries. This is true even for black smoker plumes, which typically rise only a few hundred meters above their sources as buoyant plumes (e.g., Speer and Helfrich, 1995). The long timescale of Earth’s overturning circulation is related to its strong, stable density stratification, which requires a lot of energy to be overcome. The overturning circulation is mechanically forced by turbulent mixing, with winds and tides providing most of the energy (e.g., Munk and Wunsch, 1998). Turbulence and mixing in the ocean interior is dominated by breaking internal gravity waves (e.g., Polzin et al., 1997), which therefore play a fundamental role in closing the overturning circulation.
Ocean circulation on other ocean worlds is unlikely to occur in ways that are observed on Earth. For example, on other ocean worlds, there is no expectation that a liquid surface will exist in contact with an overlying atmosphere (with the obvious exception of the shallow hydrocarbon lakes at the exterior of Titan). Hence, there will be no wind and wave action driving processes at the ocean surface. As far as overturning circulations are concerned, it is far from clear whether the density stratification on icy moons can be sufficiently strong for internal gravity waves to exist. The density stratification in any ocean is limited by the range of available densities. On Earth, both surface and bottom waters are produced by air-sea interactions at the sea surface (e.g., Talley, 2013), with gravity causing the dense waters to sink, resulting in the observed stable density stratification. In ice-covered ocean basins, the temperature at the upper interface is necessarily equal to the freezing temperature of the water (which can be perturbed significantly in the presence of salts and other dissolved constituents). While the freezing temperature of water is also pressure dependent (hence, it varies with ice topography), this pressure effect is small (e.g., McDougall and Barker, 2011) compared to the differential effects of solar radiation on Earth, implying that only a small temperature range is available for density stratification to occur. Wherever topography arises at the underside of an ocean world’s ice shell, the water density is also affected by freshwater forcing associated with freezing (where the ice is thick) and melting (where the ice is thin). Again, however, this forcing effect is anticipated to be small compared to the freshwater forcing of an ocean that is exposed to a moist atmosphere. For the oceans of Europa and Enceladus, an additional reason to expect weak density stratifications is the great depth of those oceans compared to ocean depths on Earth.
These arguments are intended to suggest that the circulations on icy moons can behave fundamentally differently from the overturning circulation on Earth. Additionally, hydrothermal plumes are also expected to be governed by different dynamics when rising in a rotationally dominated environment, which is likely to suppress entrainment and dilution once the plume has reached a limiting horizontal size (e.g., Fernando et al., 1998; Thomson and Delaney, 2001; Goodman et al., 2004). While these considerations suggest that seafloor-to-upper-interface transport on icy moons is likely very different from that on Earth, general principles of geophysical fluid dynamics still apply, even on planets with different rotation rates, different sizes and density distributions, and different forms and distributions of topography—both at the seafloor and at the ice-ocean interface.
Superimposed upon the complex, four-dimensional physical framework, we must also recognize that products (or reactants) generated at the floor (or ceiling) of any given ocean will not act as completely inert passive tracers as they are dispersed throughout an ocean. Rather, we should also anticipate that the (bio)geochemical and/or mineralogical evolution of those products may continue as they disperse, whether or not life plays a role. On Earth, one of the most surprising discoveries made in the past decade has been the recognition that chemicals released from deep seafloor vents can be transported across entire ocean basins laterally, and from bottom to top vertically (Resing et al., 2015; Fitzsimmons et al., 2017)—to the extent that fertilization of surface waters by hydrothermally sourced iron could be responsible for up to 30% of the draw-down of CO2 from the atmosphere in the Southern Ocean (Tagliabue et al., 2010). Given that such unexpected, but paradigm-challenging, processes can be revealed on this ocean world after more than 150 years of study (Thomson, 1873), we should remain open minded concerning both the trajectories and timescales over which trace materials could be transported on other ocean worlds.
One concept that could be particularly informative to deciphering other ocean systems, from our studies on Earth, is the role that dissolved-particulate interactions can play in marine (bio)geochemical cycles. On Earth, phytodetritus that originates in the sunlit upper ocean is transported through the midwater to fuel both water column and seafloor communities (Boyd et al., 2019). Another example, particularly relevant to ice-covered ocean worlds, is the dispersal of mineral-organic aggregates away from submarine vents via hydrothermal plumes (Toner et al., 2009; Breier et al., 2012; Hoffman et al., 2018). Detailed analyses of suspended and sinking marine particles (Figure 3) can record intriguing properties, leading to insights into their complex histories (Toner et al., 2015). Those insights can include their mineralogical and/or bio-inorganic origins, the formation of their own mobile redox-active microenvironments, and their capacity to adsorb inorganic tracers diagnostic of the ocean they pass through as well as its organic matter ± life.
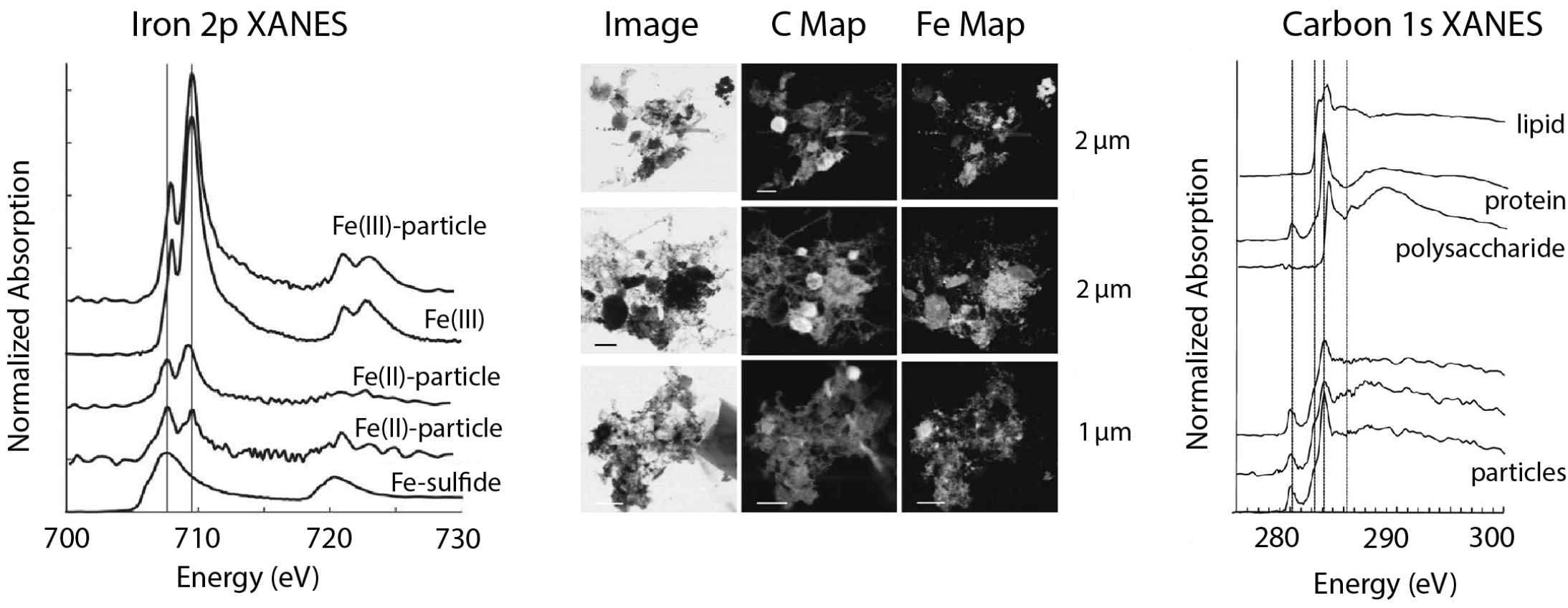
FIGURE 3. Spectroscopic analyses of suspended marine particles from a deep-ocean hydrothermal plume dispersed in seawater illustrate how mineralogical (left panel) and biogeochemical (right panel) information can be derived from individual particles to provide insights into biosignature potential. (center panel) Scanning transmission X-ray microscopy images for three particles show spatial complexity in the distribution of carbon and iron at the submicron scale. Scale bars are 2 μm in upper two rows, 1µm in lower row. Lighter colors indicate higher concentrations of C and Fe, respectively. X-ray absorption near edge structure spectra (XANES) reveal the structural form for iron (left panel) and for carbon (right panel) in the particles, where peaks of absorption at different voltages coincide with patterns observed in reference materials. For example, the particles contain peaks indicating both reduced Fe(II) and oxidized Fe(III) minerals and lipid and proteinaceous material. Images reproduced with permission from Breier et al. (2012) and Toner et al. (2015) . > High res figure
|
1 In a February 1, 1871, letter to J.D. Hooker, Charles Darwin wrote: “It is often said that all the conditions for the first production of a living organism are now present, which could ever have been present. But if (and oh what a big if) we could conceive in some warm little pond with all sorts of ammonia and phosphoric salts, light, heat, electricity, etc. present, that a protein compound was chemically formed, ready to undergo still more complex changes, at the present day such matter [would] be instantly devoured, or absorbed, which would not have been the case before living creatures were formed.” (Darwin Correspondence Project, University of Cambridge, https://www.darwinproject.ac.uk/letter/DCP-LETT-7471.xml)
Concluding Remarks
Imagine the capture of a complex, solid-phase sample derived from an interior ocean by some future NASA-funded mission to an ocean world (Figures 2 and 3). For mission concepts currently under consideration, such samples might be acquired by flying through jets of icy material expressed into space, from the fallout of such material as snow landing back onto the surface of an ocean world, or in the form of ocean waters that are otherwise expelled onto an ocean world’s surface (Mackenzie et al., 2021; Hand et al., 2022). Now imagine the drive toward deciphering the complex history of information that such a precious resource could afford. It is in anticipation of such a compelling challenge that we advocate for the application of an ocean systems science approach to support the exploration of ocean worlds.
Acknowledgments
This work is supported by NASA Astrobiology Program award # 80NSSC19K1427 Exploring Ocean Worlds: Ocean System Science to Support the Search for Life.