Introduction
The Bering and Chukchi Seas are classified by NOAA as part of Arctic marine ecosystems, and sea ice plays a critical role in structuring them. During August and September, these seas are largely ice-free; in autumn, ice advances ~1,000 km southward, reaching Bering Strait in December. Once the Chukchi Sea is frozen, extensive ice begins to form in the Bering Sea. Historically, sea ice could advance another 1,000 km, reaching the Alaska Peninsula in late winter or early spring. Together, these were the greatest seasonal ice advance in the world.
Over the last few decades, however, climate warming has reduced Arctic ice duration by ~2 days per year (Wood et al., 2015). Sea-ice timing and extent influence many components of the marine ecosystem, including ocean temperature, onset and magnitude of the spring phytoplankton/algal bloom, the ranges of subarctic and Arctic species, and the lives of the residents of more than 100 Indigenous communities who depend on the immense resources of these seas (Huntington et al., 2020).
The Bering Sea is one of the most productive marine ecosystems in the United States, supporting more than one-third of the US commercial catch of fish and shellfish ($1B for the blue economy), an extensive population of seabirds and marine mammals (including endangered species), and critical subsistence resources for Alaska’s Indigenous peoples. The Chukchi Sea hosts a productive benthic community that provides important feeding grounds for whales, seals, and walruses. NOAA plays an important role in assessing and managing the living marine resources of these seas, including various commercial fisheries, threatened and endangered marine species, and large populations of marine mammals and seabirds. To understand the impacts of climate change on these ecosystems, expansive and integrated observations are essential.
Currents over both the Bering and the Chukchi continental shelves transport heat, salt, and nutrients northward (Figure 1). Bering Strait is the only passageway for Pacific water to enter the Arctic Ocean, with an average transport of ~1 × 106 m3 s–1 (1 Sv) (Woodgate, 2018). The mass and heat transports through Bering Strait have increased over the last decade. Nearly half of this flow continues northward through Barrow Canyon into the Arctic Ocean (Stabeno et al., 2019; Stabeno and McCabe, 2020), carrying heat that contributes to the seasonal reduction in ice in the Arctic basin (Timmermans et al., 2018).
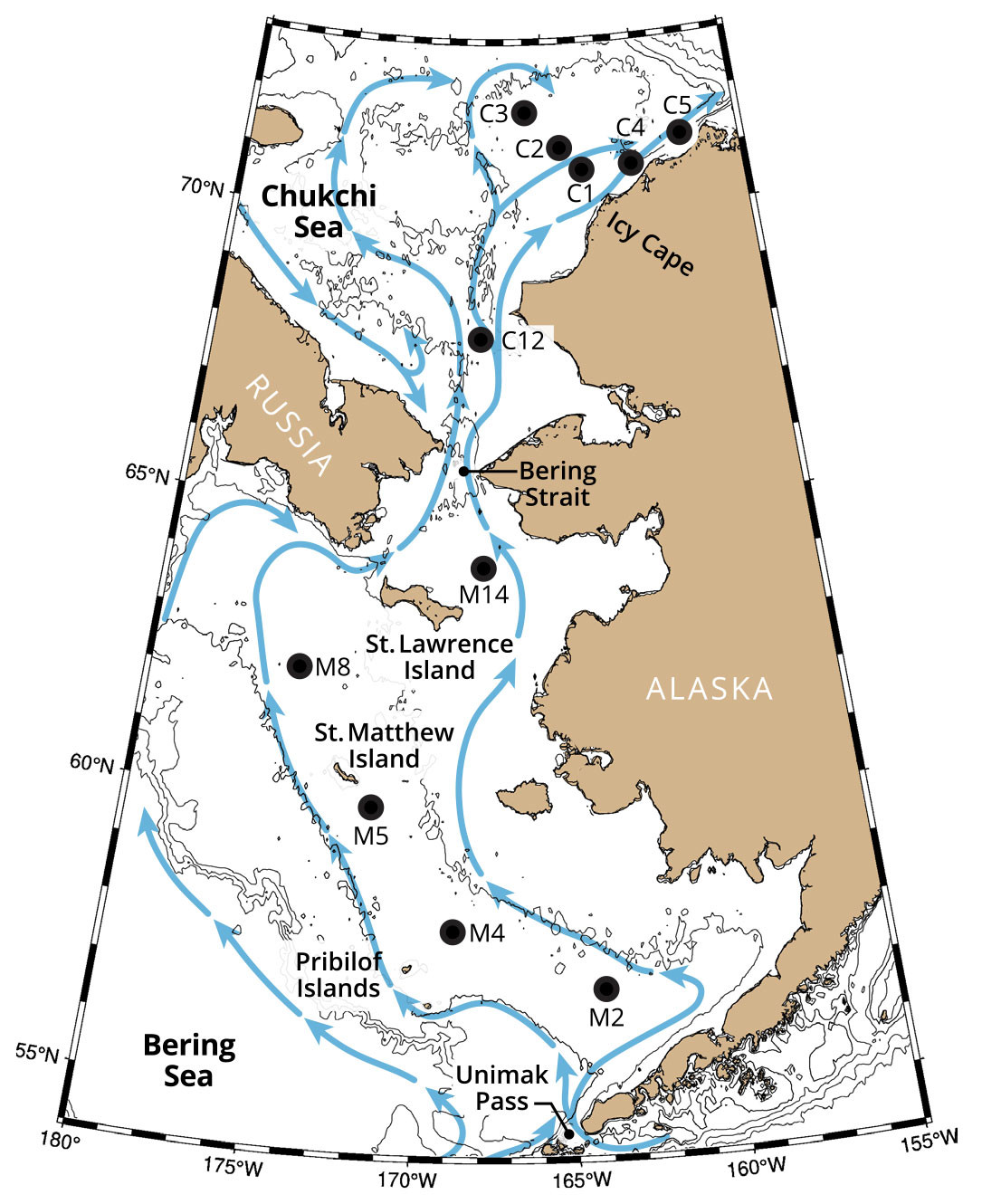
FIGURE 1. Blue arrows in this schematic show primary flow pathways in the Bering and Chukchi Seas. Labeled black dots indicate locations and names of primary mooring sites. After Stabeno et al. (2016) and Stabeno and McCabe (2020). > High res figure
|
The Bering Sea is almost equally divided between the broad eastern Bering Sea (EBS) shelf and a deep ocean basin (Stabeno et al., 2012a). The EBS shelf is divided into three cross-shelf domains: inner or coastal (0–50 m deep), middle (50–100 m), and outer (100–180 m; Coachman, 1986). In summer, the vertical structure of the coastal domain is well mixed or weakly stratified, and the outer domain consists of a wind-mixed surface layer and a tidally mixed bottom layer that are separated by a gradual thermocline (Stabeno et al., 2012b). The middle domain has a sharp two-layered water-column structure. There is also a north-south division (near 60°N), with the north having weaker tidal velocities, colder temperatures and more extensive ice, and an ecosystem dominated by benthic production. The Chukchi Sea is shallower than the Bering Sea with less distinct domains.
When frigid Arctic winds drive ice southward, the leading edge melts and thereby cools and freshens the water column (Stabeno et al., 2012b). In some years, ice can cover almost the entire EBS shelf (e.g., 2012 in Figure 2), although more recently there have been years with virtually no ice in the Bering Sea (e.g., 2018). The maximum areal ice cover in 2012 was >8.5 × 105 km2, over half a million square kilometers more than the 2018 maximum. The presence and melting of sea ice can cool the water column to below –1.7°C. As the ice retreats (or melts) and spring warming begins, the surface wind-mixed layer insulates the cold bottom water. This bottom layer, found primarily on the middle shelf, is referred to as the “cold pool.” It usually remains cold (< 2°C) until fall storms mix the water column.
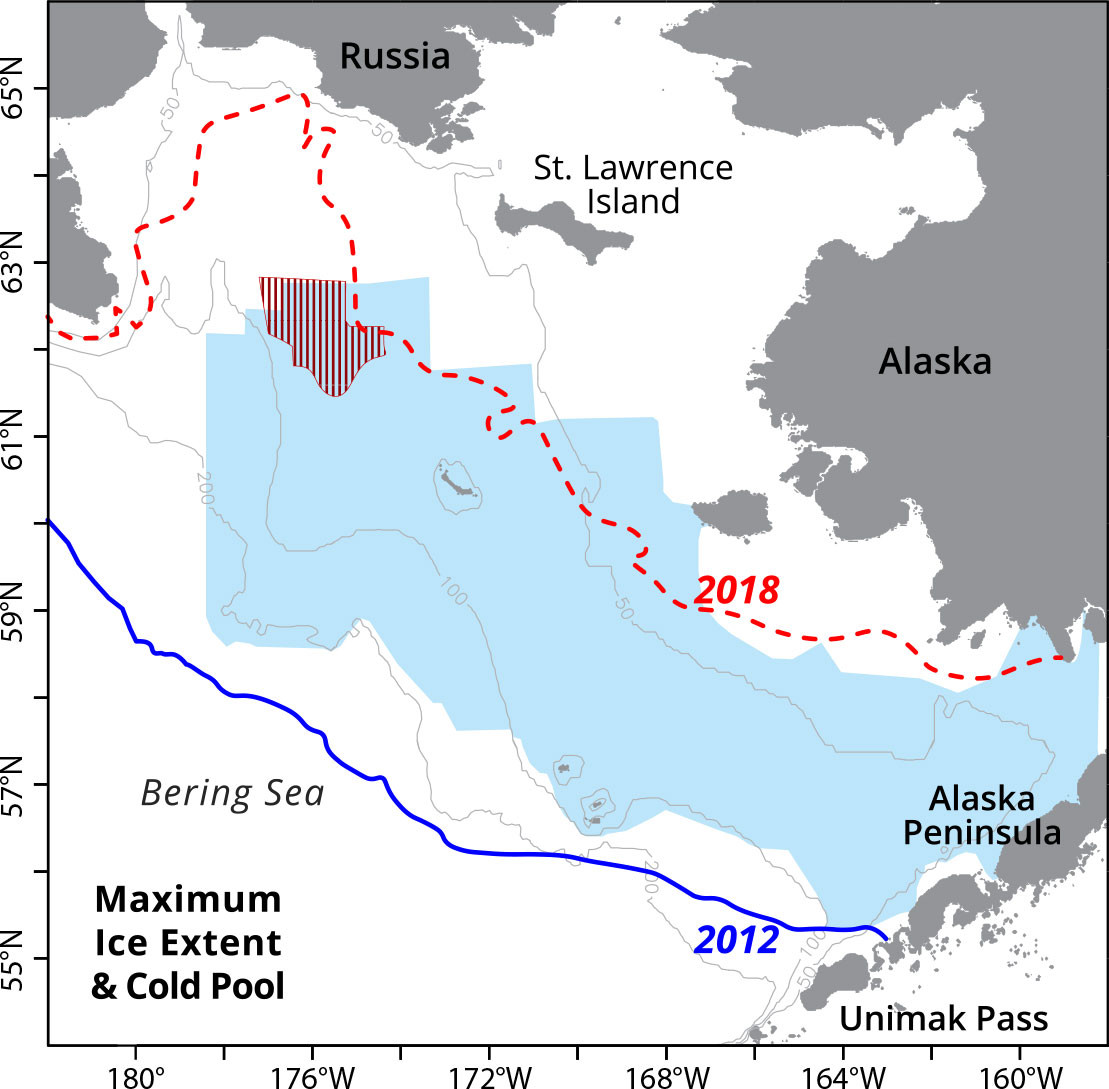
FIGURE 2. Map of the eastern Bering Sea shelf. The blue and red lines delineate the maximum ice extent in 2012 and 2018, respectively. The shaded blue and red areas outline the cold pool extents in August 2012 and 2018, respectively. > High res figure
|
The cold pool plays a critical role in structuring the Bering Sea ecosystem. For example, it provides a refuge for young-of-the-year fishes (Siddon et al., 2020), serves as a corridor for Arctic species to move southward, and forms a barrier for subarctic species. In the last five years, the limited ice extent and the associated smaller cold pool have had a strong impact on the distribution of fish and shellfish species. Alaska pollock (Gadus chalcogrammus) and Pacific cod (Gadus macrocephalus) have historically concentrated on the outer shelf and slope of the EBS, avoiding the colder (<2°C) shelf waters, but recently have been observed distributed across the shelf and even into the Chukchi Sea. In contrast, the loss of the cold pool prevented Arctic species from traveling southward and has likely contributed to the recent reduction in the snow crab (Chionoecetes opilio) population (Orensanz et al., 2004).
Working in these high-latitude seas is difficult. Even though sea ice is arriving later and retreating earlier, there remain months of ice cover (especially in the northern Bering and Chukchi Seas) when it is unwise to plan research cruises unless the vessel is ice reinforced. To better understand and forecast future changes and consequences in the Bering and Chukchi Seas, ecosystem observations are critical. Moorings of various designs, sizes, and capabilities are essential tools for collecting ocean observations during the ice-covered winter months. They include a variety of instrumentation and incorporate new and developing technology. This manuscript focuses on the evolution of long-term biophysical moorings in these high-latitude seas.
EcoFOCI Broadens its Observational Footprint Northward
Fisheries-Oceanography Coordinated Investigations (FOCI), a partnership between NOAA Fisheries and NOAA Research, began investigations in 1984 with an observational core in the Gulf of Alaska (Mordy et al., 2023, in this issue); within a decade, this work extended into the rich and varied ecosystem of the Bering Sea. Because these high-latitude seas were predicted to be much more sensitive to climate change than mid-latitude or equatorial seas, long-term observations became a NOAA priority. The Tropical Ocean Global Atmosphere-Tropical Atmosphere Ocean (TOGA-TAO) array (Hayes et al., 1991) inspired the idea of maintaining long-term moorings in the Bering Sea. In the early 1990s, FOCI’s research included a rare joint US/Russian circumnavigation of the Bering Sea (Reed et al., 1993; Cokelet et al., 1996) and several mooring deployments in the basin (Cokelet and Stabeno, 1997), but it was the highly productive EBS shelf that became the primary focus. A long-term mooring array was initiated by EcoFOCI in 1995 at mooring site M2 (Figure 1). The goal was to occupy a site with several weeks of ice cover in the majority (>95%) of years. With its weak mean currents, the middle shelf provided an ideal location for long-term measurements in the cold pool.
The first deployment at M2 ended in near disaster. A month after its March deployment, sea ice descended upon it, destroying the surface tower and dragging the mooring with its ~2,000 kg anchor ~50 km southward, where it was recovered a month later. This event instigated the deployment strategy still used at M2 today: spring deployment of the surface mooring and fall recovery of that mooring along with deployment of a subsurface winter mooring. The remaining long-term moorings in the Bering/Chukchi array (Figure 1) are all subsurface (although this is changing with new technology) and deployed for a year.
Over the following decade, the long-term biophysical mooring array was extended northward from M2 (1995) to M4 in 1999 and then to M5 and M8 in 2005 (Figure 1). During this expansion of the Bering Sea mooring array, the North Pacific Climate Regimes and Ecosystem Productivity (NPCREP) initiative was funded, expanding FOCI’s mission to officially include climate studies. FOCI and NPCREP combined to become EcoFOCI (Ecosystem and Fisheries Oceanography Coordinated Investigations), which remains a partnership between NOAA Fisheries and NOAA Research. In 2019, with the rapid changes occurring in the northern Bering Sea, a fifth long-term mooring site (M14) was established.
These long-term biophysical moorings measure the standard oceanographic variables (temperature, salinity, chlorophyll fluorescence, currents) at multiple depths. M2 also carries a meteorological package during the ice-free summer months. Since 2010, in collaboration with the University of Alaska Fairbanks, CO2 sensors have been incorporated on the summer M2 mooring. More recently, new instruments have been added to measure dissolved oxygen, nitrate, passive acoustics, photosynthetically active radiation, carbon parameters, and eDNA (see Galaska et al., 2023, in this issue). The mooring design also has been modernized; since 2016, the Prawler (Stalin et al., 2023, in this issue) has provided near-continuous profiles of temperature, conductivity, chlorophyll fluorescence, and oxygen. The newest mooring design, RISE (Refloating Ice Sensing), is a Prawler-type mooring that sinks with the arrival of sea ice and refloats the following spring with ice retreat, providing important water column measurements.
Long-term biophysical moorings in the Chukchi Sea were first deployed in 2010 with partial support from the Bureau of Ocean Energy Management (BOEM) and NOAA’s Arctic Research Program (ARP). The focus was to examine relationships among water properties, currents, and primary production, and to detect marine mammal calls. The initial deployment consisted of an array of three moorings (C1, C2, C3) off Icy Cape, Alaska (Figure 1). Over the next few years, the Chukchi array was expanded north to C4 and C5, and, more recently, south to C12. These moorings contribute to the Distributed Biological Observatory (DBO), an international ocean observatory whose goal is to detect and measure change in several biologically productive regions of the Pacific Arctic.
Bering Sea: Ocean Temperatures
On the middle shelf of the southeastern Bering Sea, M2 monitors the evolution of the water column (Figure 3). During winter the water column is well mixed. In spring/summer the surface warms by >10°C, but the bottom layer warms by only a few degrees (Figure 3c), resulting in a sharp two-layer structure. Usually in September, the water column begins to mix, becoming well mixed by late fall. Beyond the annual cycle, multi-year periods of relatively warm (e.g., 2000–2005, 2014–2017) or cold conditions (e.g., 2007–2013) are evident (Figure 3a). Cold bottom temperatures are a result of extensive sea ice (Figure 3b). When ice persists for more than a couple of weeks, the winter water column temperatures fall to below –1°C. This relationship is particularly evident in the depth-averaged temperature anomaly (Figure 3a,b).
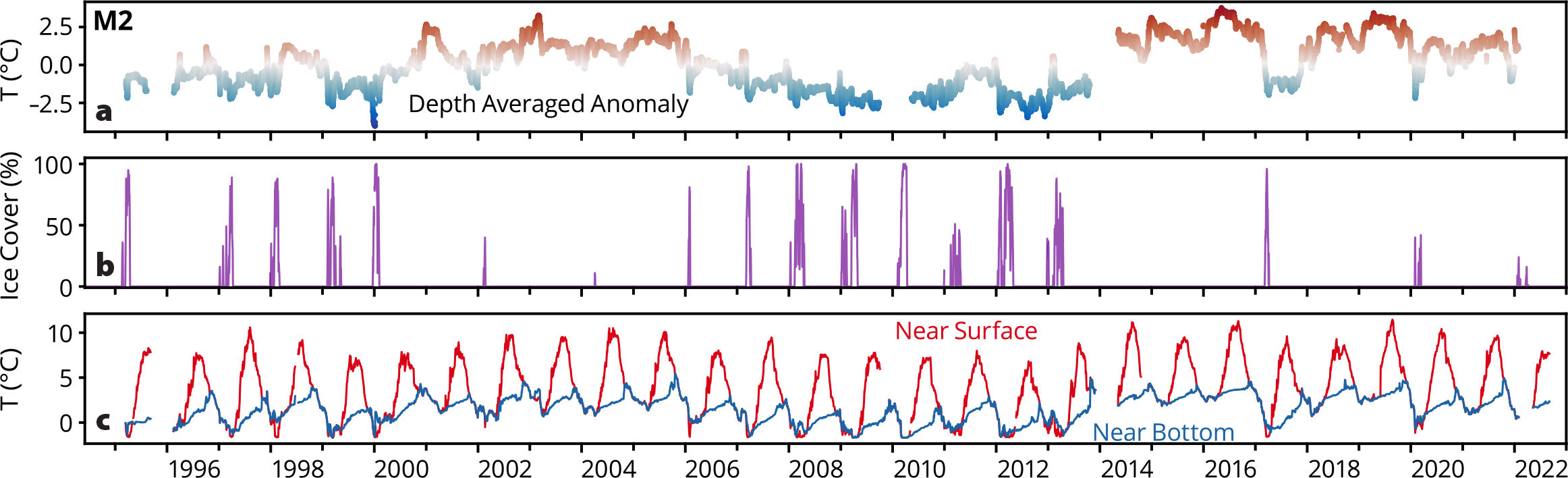
FIGURE 3. Temperature data from the M2 mooring positioned on the southern middle shelf in ~72 m of water. Near-surface (red) and bottom (blue) temperatures are plotted in the bottom panel (c), while the depth averaged temperature anomaly from the long-term mean is shown in the top panel (a). The middle panel (b) shows the average ice cover in a 50 km × 50 km box centered on M2. Percent ice cover is from the National Snow and Ice Data Center (NSIDC), Bootstrap from 1995 to 2021 (Comiso, 2017), and Near-Real-Time in 2022 (Meier, 2021). > High res figure
|
One long-standing expectation was that as the Bering Sea warmed, subarctic species such as pollock and Pacific cod would expand their ranges over much of the EBS, thus increasing abundance. In 2001, this proved not to be the case. Rather, the absence of ice algae and a later phytoplankton bloom caused a reduction in zooplankton abundance and thus pollock survival, which resulted in a halving of the commercial fishery pollock quota from 2003 to 2008. The return of more extensive ice cover and colder conditions in 2006 brought improved recruitment of pollock. An interesting turn of events occurred with the return of warm conditions in 2014—pollock and Pacific cod did indeed spread across the EBS and even into the Chukchi Sea. This sharply different response was a result of conditions in the northern Bering Sea.
The northern Bering Sea temperature observations at M8 exhibit a similar pattern to that at M2 (Figure 4). There are indications that in M8’s first year of deployment (2005), ocean temperatures were warmer with less ice. As at M2, there was a transition to reduced ice and warmer ocean temperatures (especially bottom temperatures) in 2014. This warming had, and continues to have, a large impact on the ecosystem. Adult pollock and Pacific cod are found in temperatures above ~0°C (Eisner et al., 2020). Prior to 2014, there were usually <10 days at M8 when bottom temperatures rose above –0.5°C (2005 had ~50 days); after 2014 (excluding 2015), there were 100 to 200 days when bottom temperatures remained above –0.5°C. Migration corridors for subarctic fishes (e.g., cod) require stable thermal routes (Stensholt, 2001), which these warmer temperatures provide.
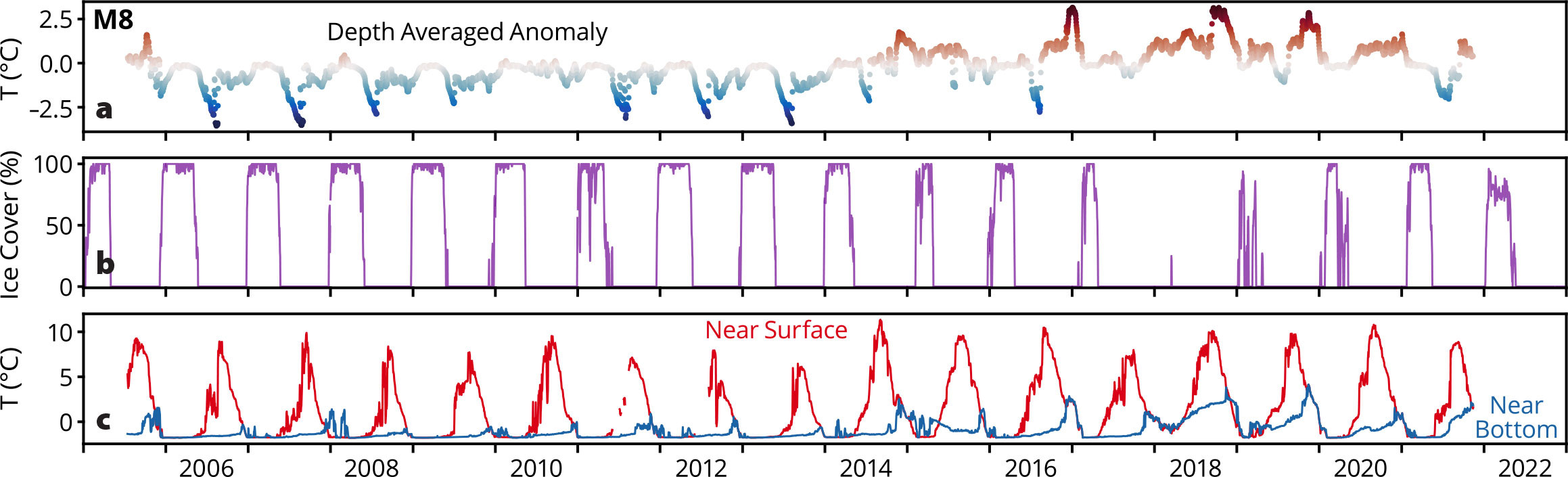
FIGURE 4. Plots are shown as in Figure 3, except for site M8 rather than site M2. > High res figure
|
Chukchi Sea: Ocean Temperatures
Of the three moorings at Icy Cape (C1, C2, and C3), C2 was identified as the primary site, and a mooring has been deployed there each year since 2010. The water at C2 is shallow (~43 m depth), and ice keels as deep as 30 m have been measured at the nearby mooring C1 (Stabeno and McCabe, 2020). Thus, to keep moorings safe in the ice-covered winter months, instruments and flotation are within 10 m of the seafloor. More recently, new technology has allowed measurements in the upper 30 m of the water column. In 2019, a RISE mooring was deployed at C2, sinking with the arrival of ice and resurfacing the following summer after ice retreated to provide critical late spring and summer water column data.
The limited time series of near-surface observations compare well with satellite-derived SST (Figure 5). The pattern of warmer Bering Sea temperatures is also evident at C2, although warming starts a year later (2015 instead of 2014) for these waters, which flow northward into the Arctic basin. Interestingly, these shelf waters are more saline and thus denser than near-surface water in the Arctic basin (Stabeno and McCabe, 2020), so they sink as they exit the shelf, introducing warmer water below the surface. This warmer water affects sea ice, delaying freeze-up and reducing ice thickness.
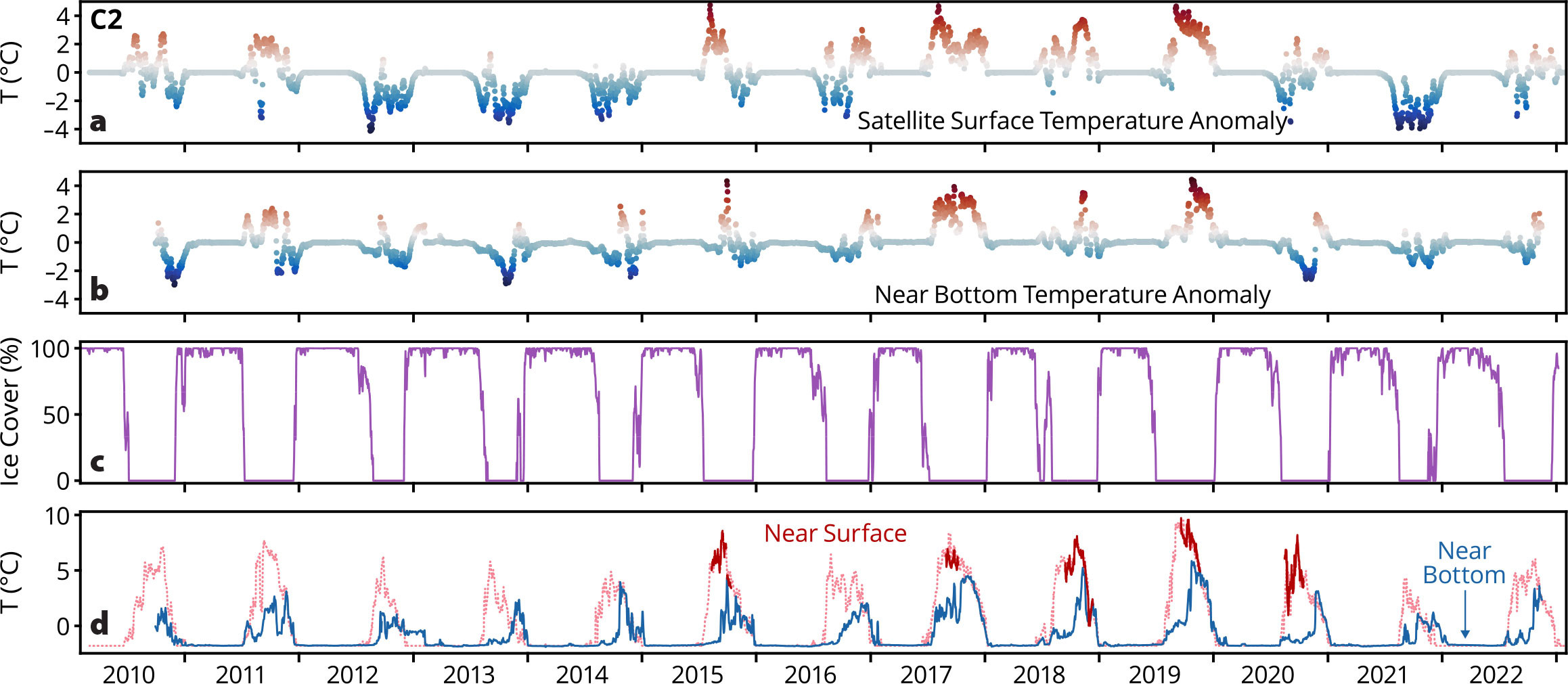
FIGURE 5. Time series of temperature from the C2 mooring site in the Chukchi Sea. Near-surface (red) and bottom (blue) temperatures are shown in the bottom panel (d). The pale red record is satellite-derived sea surface temperature from the NASA JPL MUR product, which compares well (r2 = 0.76, 1.05°C offset) with near-surface instrument records (dark red). Temperature anomalies from the long-term means of the near-surface (a) and near-bottom (b) records are plotted in the top two panels. The lower middle panel (c) shows the average ice cover in a 50 km × 50 km box centered on C2. > High res figure
|
The warmest year occurred in 2019. One observation from C2 is that bottom temperatures were greater than –0.5°C for approximately 60 days each year in 2010–2014, and, except for 2021, that pattern has continued in recent years (Figure 5). These warmer bottom temperatures provide a survivable habitat during summer for subarctic fishes that have migrated north from the Bering Sea. The question remains, does the bottom water in the Chukchi Sea cool slowly enough for these fishes to retreat back to the Bering Sea to overwinter?
The Impact of Climate Change on the Bering-Chukchi Ecosystems
Continuous moored observations of the rapidly changing Bering-Chukchi ecosystems have never been more important. The EcoFOCI long-term mooring array enables direct quantification of the rapid biophysical changes currently underway at the base of the ecosystem, thereby providing an essential context for additional ship-based observational snapshots and modeling studies.
The Bering Sea is undergoing a reduction in sea-ice extent and duration, with the lowest extent on record occurring in 2018 (Overland, 2022), resulting in warmer ocean temperatures. Such physical extremes were due to positive feedbacks from warm air and sea temperatures, sea-ice loss, southerly winds, and a wavy jet stream (Stabeno and Bell, 2019), ultimately leading to loss of snow crab habitat, reduction of northern salmon runs, increased mortality for seals and seabirds, and occurrence of harmful algal blooms (Siddon et al., 2020).
Sea ice duration is also decreasing in the Chukchi Sea, although not its extent because it remains ice covered in winter. The flow in the Bering and Chukchi Seas brings warmer water northward, resulting in earlier ice retreat and later ice arrival in the Chukchi Sea (Stabeno et al., 2019). The mooring array off Icy Cape provides data to quantify total mass (~0.5 Sv) and heat transport into the Arctic basin (Stabeno and McCabe, 2020). The flow of Pacific water through Bering Strait is increasing as is the heat flux. The northward flowing Pacific water is also an important source of nutrients in the Chukchi Sea (Mordy et al., 2020). Advection, the reduction in sea ice, and a warming ocean have impacts throughout the ecosystem.
Sea ice plays a role in determining the timing of the spring bloom. Ice algae within and under the ice begin to bloom in early spring. Zooplankton graze on the algae underneath the ice and near the seafloor (Stabeno et al., 2020). As the ice melts, much of this ice algae falls to the seafloor, where it supports the benthic community in the northern Bering and Chukchi Seas through a process called pelagic-benthic coupling (Grebmeier, 2012). Without sea ice, open-water phytoplankton blooms dominate, supporting a more pelagic ecosystem (Hunt and Stabeno, 2002; Frey et al., 2022).
The timing of sea-ice formation and retreat greatly impacts phytoplankton growth and therefore CO2 fluxes. Rapid warming concomitant with reduced sea-ice coverage is causing the regional summer CO2 sink to decrease (DeGrandpre et al., 2020). Recent results suggest that spreading of corrosive conditions into the Pacific layer of the Arctic halocline is likely driven by anthropogenic CO2 (Qi et al., 2022), with the cold Pacific Winter Water serving as one important source. The increased corrosiveness of this water results from cold temperatures and from the considerable amount of biological respiration that takes place in the northern Chukchi Sea. However, many questions remain, such as how ongoing warming and changes in sea ice may alter the region’s algal bloom timing, magnitude, and composition and how those changes will ultimately impact the downstream biogeochemistry, including CO2 fluxes and ocean acidification.
In the Bering and Chukchi Seas, zooplankton populations vary with differing ice conditions. For instance, from 2002 to 2018, Calanus spp. were largely absent during years without spring ice, with the opposite occurring in years when ice persisted into spring. Just as the distribution of fish varied between the two warm periods, so did zooplankton populations (Kimmel et al., 2023), with increased abundances of small copepods and decreased abundance of the larger Calanus spp. in the later warm period.
In addition to warmer waters allowing subarctic fish species to expand their ranges northward, these conditions are also impacting the ranges of marine mammals. For instance, most bowhead whales (Balaena mysticetus) in the US Arctic feed in the eastern Beaufort Sea in summer and overwinter in the northern Bering Sea (Clark et al., 2015). In years when sea ice extends into the southern EBS, bowhead calls are detected (via passive acoustics at the mooring sites) as far south as M2. Recently, with rising ocean temperatures and reduced sea-ice extent, bowheads have been overwintering north of Bering Strait (Szesciorka and Stafford, 2023), and in 2018–2019, some were observed overwintering in the eastern Beaufort Sea (Insley et al., 2021).
The eastern population of North Pacific right whales (Eubalaena japonica) are critically endangered (N ~ 30). They range more broadly over the EBS during warm years than they do in cold years (Zerbini et al., 2015), with recent detections in the northern Bering Sea (Wright et al., 2019). If this expansion continues into the Bering Strait region, the risk of ship strikes within this choke point will increase (Huntington et al., 2020); the loss of even one individual of this population would be detrimental to its recovery.
Contributions to NOAA’s Mission and the Future
EcoFOCI mooring observations, among the longest near-continuous data sets in US waters, are supplemented with ship-based discrete measurements that include parameters not easily monitored on moorings. Together these observations have expanded our understanding of these ecosystems, are used by researchers and stakeholders, and inform important living marine resource management decisions (e.g., Stock Assessment and Fishery Evaluation Reports for Bering Sea; Mordy et al., 2023, in this issue).
The future of the NOAA Pacific Marine Environmental Laboratory (PMEL) component of EcoFOCI will focus on enhancing and expanding long-term moorings. Through innovation and partnerships within NOAA and beyond, the program is advancing NOAA’s observing capacity with improved technologies for ice-influenced environments. Long-term observations will continue at the biophysical mooring sites (five in the EBS, six in the Chukchi Sea, three in the Gulf of Alaska). PMEL and Alaska Fisheries Science Center components of EcoFOCI will continue hydrographic and zoo- and ichthyoplankton sampling efforts on research cruises in the Gulf of Alaska and the US Arctic to augment this observational network (see Figure 1 in Mordy et al., 2023, in this issue).
These observations also contribute to the NOAA-wide effort to inform, improve, and increase use of models to enable ecosystem forecasts. For example, detecting the pulse nature of seasonal inflow of water on the Northeast Chukchi shelf was influential in development of the conceptual Arctic Marine Pulses model (Moore et al., 2018). New instrumentation in the form of pop-up floats (Stalin et al., 2023, in this issue) has already provided a unique solution to mapping the time evolution of the cold pool in the Bering Sea and has expanded our understanding of the role ice algae plays in Chukchi Sea benthic ecosystems (Stabeno et al., 2020). Observations that have increased our understanding of these rich and valuable ecosystems are used to guide and validate EcoFOCI models (Hermann et al., 2023, in this issue).
EcoFOCI will continue to incorporate new and emerging technologies into field, laboratory, and modeling research, and expand data management to improve our understanding of Alaskan marine ecosystems and the impacts of a changing climate. The mechanistic understanding provided will enable NOAA and its stakeholders to better understand, predict, and mitigate the impacts of climate change and other anthropogenic influences on these valuable ecosystems.
Acknowledgments
The success of EcoFOCI is a direct result of the dedication, hard work, and insights provided by an extensive list of personnel who did the many jobs necessary to conduct oceanographic research. We owe our current understanding of the oceanography and ecosystem structure in Alaskan marine waters to their efforts. We thank and acknowledge NOAA, including Global Ocean Monitoring and Observing Arctic Research Program (FundRef https://doi.org/10.13039/100018302), and the other agencies (e.g., US National Science Foundation, US Bureau of Ocean Energy Management, the North Pacific Research Board, and the State of Alaska) who have supported our extensive monitoring network over the past 30 years. This publication is partially funded by the Cooperative Institute for Climate, Ocean, and Ecosystem Studies (CICOES) under NOAA Cooperative Agreement NA20OAR4320271. This is PMEL contribution 5480, EcoFOCI contribution 1037, and CICOES contribution 2023-1297.