Introduction: Ocean World Observations from Remote to In Situ
The last few decades of planetary exploration have revealed that Earth is but one among several ocean-bearing worlds in our solar system, and these “ocean worlds” are candidate targets for missions designed to seek evidence of life beyond Earth (Hendrix et al., 2019). Our growing understanding of these worlds is a function not only of the observations made there but also of the rich interpretive context provided by our understanding of physical, chemical, and geological processes on our own ocean world, Earth. As the objectives of ocean world exploration turn increasingly toward life detection, our knowledge of biology in Earth’s ocean, and the past and present observing strategies that underlie it, can be an equally valuable guide. Indeed, our perception of the habitability of these distant oceans has evolved with the growing understanding of both the nature and diversity of ocean worlds in the outer solar system and the diversity and capabilities of life in our own ocean. For example, the first signs of an ocean world in the outer solar system came with Voyager’s 1979 flyby of Jupiter’s moon Europa, scarcely two years after the discovery of hydrothermal vents on Earth. Observations made in Earth’s ocean have taught us that, with very few possible exceptions, life is found under almost all physicochemical conditions present there. In turn, these findings support optimistic views about the distribution of life on other ocean worlds.
Planetary exploration, including that of our own planet, takes place through campaigns of targeted observations and missions, each of which builds upon previous findings. The methods of data acquisition and often the scales of observation evolve through successive campaigns as enabling technologies and the understanding of the target environment advance. For example, the exploration of Earth’s ocean has expanded from ship-based campaigns to crewed and remote submersibles, subseafloor drilling, and air- and space-based observations. In parallel, the scales of observation have expanded from naked eye to microscopic and global. The exploration of Mars has also involved a progression, in its case, from global to microscopic scales of observation. From the hand-drawn telescope images of Schiaparelli, observations have evolved through increasingly powerful telescope imagery and spacecraft-based remote sensing to landers and rovers performing in situ imaging and analysis at landscape to microscopic scales.
The observations now being made at Mars will, in turn, inform the careful selection and return of samples that can then be prepared and analyzed on Earth with a broad range of methods that are presently not viable to conduct in situ. This progression from remote to in situ, and from telescopic to microscopic scales of analysis, reflects an opportunity inherent in any exploration strategy: to optimize the potential for scientific advancement by leveraging observations made at one scale to formulate new objectives, drive new enabling technology, and inform the targeting, implementation, and measurement strategies that are deployed in successive observing campaigns. Such optimization will be essential as we begin to pursue the bold objective of detecting evidence of life beyond Earth.
The early characterization of the outer solar system through remote-sensing methods has provided insights into its geophysics, surface morphology, and chemistry. As the objectives for further characterizing these worlds begin to focus on habitability and life detection, the mode of study is shifting in parallel to orbital/flyby and landed in situ observing campaigns. For technological reasons, the first generation of missions to explore the surfaces of contemporary ocean worlds will likely not be mobile, and sample acquisition systems may be limited to depths of tens of centimeters (e.g., see Hand et al., 2017). These constraints will require that we optimize our potential for detecting evidence of life through well-informed selection of landing sites and well-informed choices of how best to select, acquire, and analyze samples. Prior observations made at these worlds inform our understanding of physical and chemical processes at planetary to regional scales. To leverage this information in choosing where to land, how to sample, and what observations to make, we must combine it with an understanding of how the distribution of life and its products relates to underlying physical and chemical controls across a range of scales.
Our understanding of Earth’s biosphere is an invaluable resource for informing the search for life on ocean worlds. We understand the distribution of life on Earth as a system-level phenomenon—one governed by interacting physical and chemical processes—across scales ranging from microscopic to global. We have been able to study all hydrosphere surface environments using the full range of methods that could be applied in the search for life on ocean worlds, from remote hyperspectral satellite imaging and radar sounding to in situ chemical analysis and microscopy. Complementary numerical modeling and laboratory analyses have informed us as to the possibilities for life’s origin on Earth, how certain environments became and remain habitable, and how surface features reflect underlying processes that influence not only the physical and chemical land- or seascape but also the distribution of life across it. “Ground truth” data sets, which correlate aerial or space-based remote-sensing data with structural, chemical, and biological characterization of surface and subsurface materials, are especially critical. Leveraging these data sets, the methodology developed to obtain them, and the understanding built upon them, will help to place spacecraft observations within an interpretive framework that links biological processes to physical and chemical ones. Doing so will inform the selection of landing sites based upon remote observations and also help to identify optimal sampling and measurement strategies. Here, we explore the synergies between ocean system science on Earth and the pursuit of life detection objectives on other ocean worlds.
The Distribution of Biology and Biogenic Materials on Earth
On Earth, life and biogenic materials are heterogeneously distributed according to the availability of resources, the strength of stressors, and various physical transport processes. A useful framework for understanding the distribution of potential biomass in any ecosystem is the balance between metabolic opportunities and environmental stressors (Shock and Holland, 2007). Higher stress incurs an increasing cost on cellular physiology, which can only be met if there is sufficient free energy in the system. The excess energy that remains after basic cellular maintenance is met (i.e., repairing damage caused by environmental stress) is available for growth. The availability of nutrients and carbon substrates (organic for heterotrophic life, inorganic for autotrophic life) further controls biomass, as do physical and ecological processes of attrition, such as grazing. The varying balance of these factors results in a heterogeneous distribution of life and biogenic materials at multiple spatial scales (Figure 1).
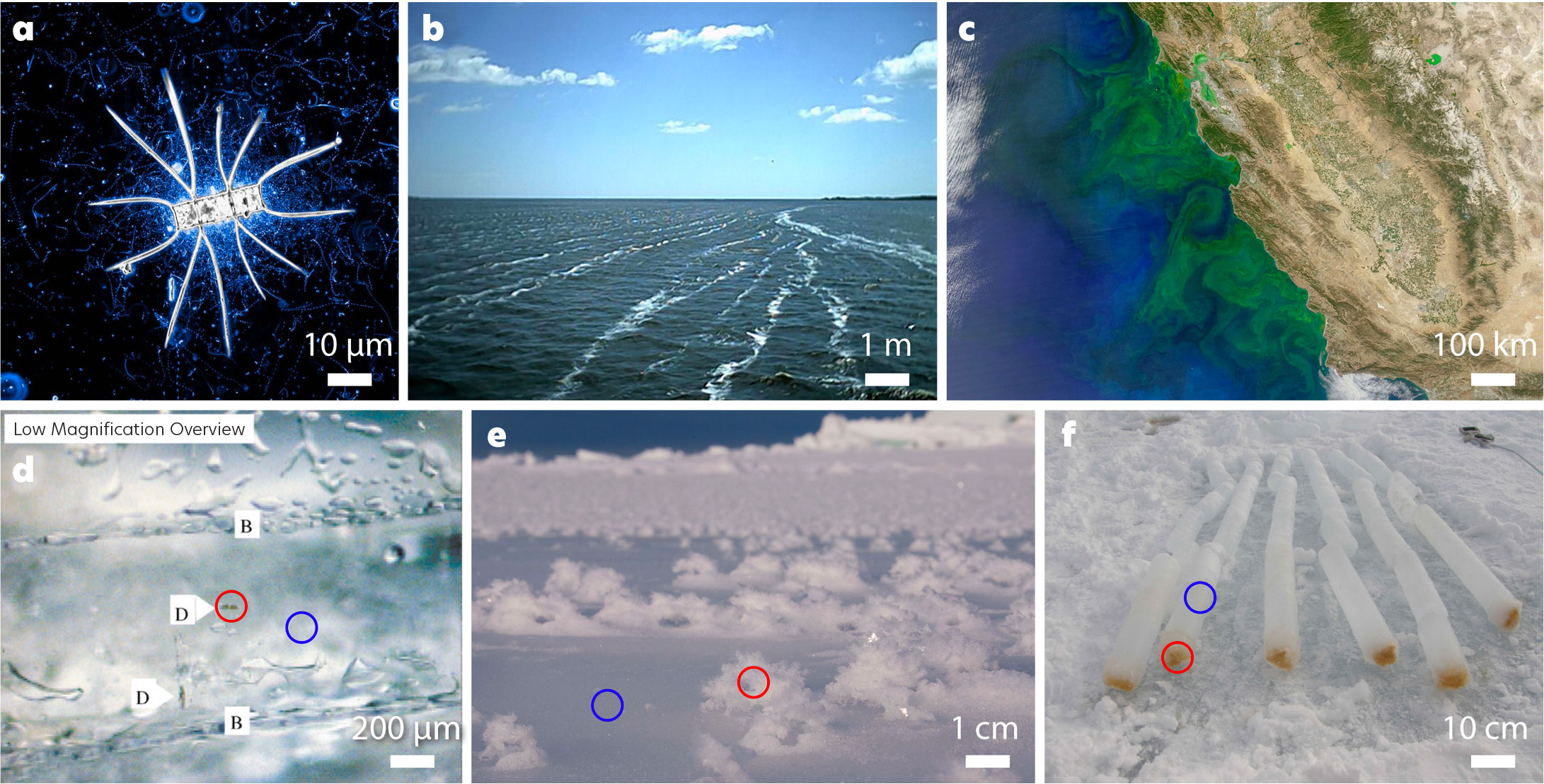
FIGURE 1. Distributions of biomass at different spatial scales in ocean ecosystems. (a) Gradients of carbon around autotrophic cells create strong spatial patterns among heterotrophic bacteria (https://ki-galleries.mit.edu/2015/diatom; credit Steven Smriga, MIT, courtesy of the Koch Institute Image Awards). (b) Langmuir circulation creates zones of high and low biomass on the surface (https://www.ldeo.columbia.edu/~ant/Langmuir.html; credit Andreas Thurnherr, LDEO). (c) Nutrient concentrations lead to strong gradients in biomass between onshore and offshore locations (https://oceancolor.gsfc.nasa.gov/gallery/658/). Panels d, e, f illustrate the distribution of biomass within the sea ice system at different scales. Zones of high biomass (red circles) can be found adjacent to zones of low biomass (blue circles). (d) Single ice algal cells are partitioned into brine pockets within sea ice, leaving the ice crystals with relatively low biomass (Krembs et al., 2002). (e) Frost flowers are nearly ubiquitous on the surface of newly forming sea ice and contain an order of magnitude more bacteria than adjacent sea ice surfaces (Bowman and Deming, 2010). (f) In a classic example of the stress vs. resource trade-off, sea ice algae concentrate at the base of sea ice cores collected from McMurdo. > High res figure
|
The distribution of biology in sea ice, which is frequently referenced as a proxy for certain ice types on ice-covered ocean worlds, exemplifies the influence of physical and chemical processes on biological heterogeneity across scales (Figure 1d–f). Although sea ice differs from these environments in that energy is provided primarily by sunlight vs. chemical energy sources (e.g., Chyba, 2000), how life is organized in that system is not fundamentally different from any ice environment with an exogenous energy source. Within the sea ice system, life is partitioned at submillimeter scales into brine pockets that form as salt and particles are excluded from ice crystals during sea ice growth (Krembs et al., 2002). A microscale evaluation of a mature sea ice crystal would report a feature nearly devoid of life, while a similar evaluation of an adjacent brine channel would find a rich microbial habitat. Even within the brine channels, life is distributed non-uniformly over centimeter scales, both vertically and horizontally. For example, mature sea ice typically exceeds one meter in thickness. Further toward the surface of this ice, the temperature is colder; the in situ salinity is higher; stressors, such as ultraviolet light, are more severe; and connectivity with the external environment is reduced (Ewert and Deming, 2013). As a result, despite a considerably greater supply of energy (sunlight), there is no growth of photosynthetic biomass (Arrigo, 2014). However, the importance of physical transport in biomass accumulation is seen in the exclusion of brine as ice crystals form, and its subsequent deposition on the ice surface. The moisture evaporates and quickly super-saturates in a cold atmosphere, freezing out on available nucleation sites. Once a crystal is nucleated, it encourages further nucleation, leading to patches of fragile ice features known as frost flowers. Through a process still not fully understood, these features accumulate additional salt and bacteria, leading to a several-fold accumulation of biomass over nearby ice surfaces (Bowman and Deming, 2010).
The distribution of life in sea ice exemplifies the myriad ways in which physical processes can create heterogeneity across multiple spatial scales. Understanding the balance of factors that underlie such heterogeneity, and projecting that understanding into the context of environments beyond Earth, can help to minimize the chance of false negative results (i.e., failure to detect evidence of life that is actually present). Heterogeneity at meter and larger scales determines where a lander should be placed. At smaller scales, it determines the dilution of signal that occurs when a scoop, rasp, saw, or other collection device integrates over the volume of a sample.
It is important to recognize that the distribution of biological materials may differ from the distribution of biomass. Again, Earth’s ocean provides useful examples of how biological molecules—including biomarkers—are organized. Biological molecules are not distributed homogeneously but rather are at their highest concentration near their sources (this is what drives the distribution of heterotrophic biomass; note the light blue material in Figure 1a). This principle accounts for the phycosphere, or zone of chemical influence, around autotrophic cells in aqueous environments (Amin et al., 2012). To the extent that these molecules are more persistent in the environment than the organisms that created them, and more easily transported, they are more uniformly spread. Large heterogeneities in biomass distribution create enhanced potential for detection in areas of high biomass concentration and, simultaneously, for false negative results in corresponding areas of low concentration. It thus represents both challenge and opportunity for life detection efforts and also underscores the need for targeting and observing strategies that are carefully informed by an understanding of the processes that control biomass distribution.
Key Differences between Earth and the Ice-Covered Ocean Worlds
Understanding the balance of factors that underlie the heterogeneous distribution of life and its products across Earth’s hydrosphere provides a conceptual framework to guide the search for evidence of life on other ocean worlds. At the same time, it is important to acknowledge that Earth differs from these other worlds in ways that significantly impact this balance and therefore the nature and abundance of evidence for life that our world presents to the observer.
Resource Availability
Synthesis of new biomass (productivity) can be limited by availability of energy, nutrients, or in the case of autotrophs, the reducing power needed to “fix” inorganic carbon into organic compounds, whereas maintenance of existing biomass depends on energy availability. On Earth, the flux of light directly into liquid water habitats provides such an abundant energy source that the oceanic biosphere overall is limited by nutrient fluxes rather than energy (Dutkiewicz et al., 2006). As an ocean-wide average, only about 7% of incident, biologically usable light energy is absorbed by photosynthetic organisms (Field et al., 1998). Deeply ice-covered ocean worlds would lack this large flux of energy, and any life therein would depend instead on chemical energy fluxes supplied by geochemical and/or radiolytic processes. Estimates of such flux for Europa and Enceladus (e.g., Vance et al., 2016), per unit volume of their respective oceans, are at least seven orders of magnitude lower than the flux of solar energy entrained into photosynthesis per unit volume of Earth’s ocean (Hand et al., 2017). Beyond energy, vigorous continental weathering on Earth drives riverine and aeolian fluxes of limiting nutrients, such as P and Fe, that may also be orders of magnitude higher than could occur on ice-covered ocean worlds (Lingham and Loeb, 2018). The implication of these potentially large differences in resource fluxes is that, on a global basis, both the magnitude of productivity and the standing biomass that can be supported may be greatly diminished on the other ocean worlds relative to that on Earth. It is essential that our measurement strategies factor in this diminished potential.
Stressors and Attrition
Because organisms and their biochemical remains are, themselves, sources of energy and nutrients for other organisms, biological consumption of these resources is a key control on the abundance and distribution of life and organic compounds in Earth’s ocean. For example, while the net productivity (rate of new biomass synthesis) of land-based and oceanic photosynthesis is approximately equal (Field et al., 1998), the standing photosynthetic biomass in the ocean is estimated to be about 500-fold smaller than that on land (Bar-on et al., 2018)—a difference attributable primarily to rapid grazing and the relatively fast growth rates of microbial primary producers in the oceanic case. One consequence of rapid biological cycling (production/consumption) is that associated biomass and some dissolved biomolecules have short lifetimes. For example, the residence time (Box 1) of both phytoplankton (Field et al., 1998; Falkowski and Raven, 2007) and biologically produced compounds such as amino acids (Moran et al., 2016) can be as short as hours to days in the productive regions of Earth’s ocean. Systems characterized by orders-of-magnitude lower productivity, as is likely the case for ice-covered ocean worlds, will differ markedly. There, lower productivity would necessarily translate to correspondingly longer residence times or lower concentrations of biomass/biomolecules, or a combination of the two (see Box 1). The implication of much longer residence times is that abiotic degradation and transport processes that are negligible in an Earthly context may be more important as controls on the nature and abundance of evidence for life on other ocean worlds. For example, racemization—the abiotic interconversion of the D and L forms of amino acids—occurs over timescales of tens to hundreds of thousands of years at temperatures characteristic of Earth’s ocean (Bada, 1982). Such a process is inconsequential in affecting the ratio of D and L forms, a potential indicator of life, when the residence time of amino acids is in the range of centuries or less but would severely degrade such evidence when residence times are orders of magnitude longer.
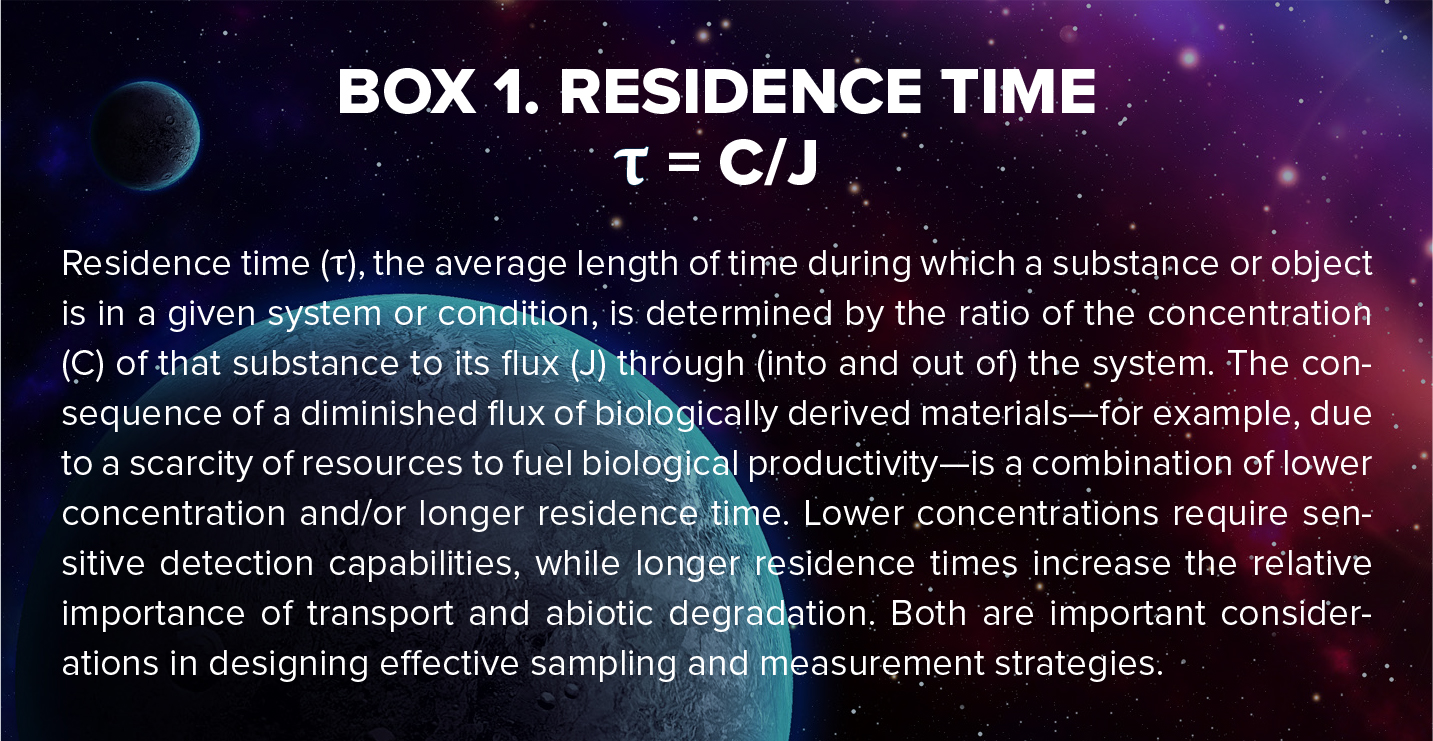
|
Physical Transport
Earth’s ocean potentially exhibits more vigorous transport and mixing than might occur on ice-covered ocean worlds. Wind-driven circulation in the surface ocean can occur with velocities up to the meter per second range (e.g., in the Gulf Stream and Kuroshio currents), and associated turbulence serves to mix these surface waters both laterally and vertically on relatively short timescales. Moreover, evaporation and/or cooling of surface fluids leads to density-driven (thermohaline) circulation that cycles water through the deep ocean on a timescale of about 1,500–2,000 years. These processes exert an important control on the flux of resources to life in Earth’s ocean. For example, wind-driven circulation causes upwelling of nutrient-rich deep waters that sustain the high levels of productivity and abundant biomass observed in Earth’s richest fisheries (Figure 1c). Ice-covered worlds lack the potential for wind-driven circulation and, while crystallization/melting of ice and hydrothermal flow can impart density differences, the much lower gravity of these worlds will lead to correspondingly lower density-driven flow rates. On the other hand, ice-borne transport of materials, potentially including materials both required and produced by biology (Wolfenbarger et al., 2022), may be more prevalent and important on fully ice-covered worlds than on Earth. Our sampling and analysis strategies should be informed by improved understanding of the potential for physical processes to both (re)distribute resources and transport evidence of life from sites of production to sites of sampling.
These and numerous other differences will significantly impact the nature, abundance, and quality of evidence for any life that may exist on other ocean worlds, relative to what we observe in the world around us. It is critical to be mindful of these important differences as we develop strategies to seek evidence of life beyond Earth.
Leveraging the Science and Technology of Ocean System Research
The exploration of ocean worlds elsewhere in our solar system can leverage not only our understanding of Earth’s ocean system but also the methodology used to develop that understanding and knowledge of the diversity of environments and processes from which it derives.
Earth’s hydrosphere comprises a range of environments that can serve as natural laboratories to test and refine our understanding of processes potentially at work on other ocean worlds, including those that may shape the context for life. For example, the exploration of terrestrial lakes beneath thousands of meters of glacial ice guides our understanding of sub-ice oceans and perched water bodies theorized to form in the outer solar system (e.g., Schmidt et al., 2011; Dombard et al., 2013; Michaut and Manga, 2014), and the objectives and measurement approaches that may be used to study them. Recent exploration of two lakes beneath roughly a kilometer of ice in West Antarctica reveals inhabited environments that differ greatly in their geochemistry, including dissolved oxygen levels (Davis et al., 2019; Skidmore et al., 2019). Understanding how organisms “make a living” under these differing conditions can inform what we search for in prospective low oxygen oceans (perhaps on Enceladus; Glein et al., 2015) and higher oxygen oceans (perhaps on Europa; Hand et al., 2007; Russell et al., 2017) in the outer solar system. More broadly, the hundreds of known subglacial lakes provide a diverse array of natural laboratories, with various geochemical and geothermal inputs, for investigating the diverse ways that life may function in cold, non-photosynthetic, but variously oxygenated environments (Siegert et al., 2016; Livingstone et al., 2022).
Even as we seek terrestrial environments that approximate the conditions of icy ocean worlds, it is important to acknowledge that there are no perfect analogs. In using these environments as observational points of reference, we must also recognize key differences and account for how they may propagate through multiple levels of the system. For example, the development of models to understand fracturing and fluid transport within ocean world ice shells can build on observations of ice dynamics in terrestrial settings (e.g., Walker and Schmidt, 2015; Craft et al., 2016), but these models must also account for the differing dynamics of colder and potentially much thicker ice. Similarly, we must recognize and account for differences at the physical or chemical level that, as described above, may propagate into the abundance and distribution of life. For example, the habitable niches observed at the ocean-ice interface on Earth receive trace amounts of sunlight that fuel the biology there, and the physical and chemical dynamics that drive distinctive patterns in the distribution of life play out in specific regimes of temperature and pressure. We must expect and account for differences that may arise when the fluid-ice interface lacks the input of light energy, or when ice crystals form dissimilarly under the differing physical and chemical conditions that are likely present in ocean world ice shells. Even here, terrestrial environments can provide an important resource. By identifying analogs for specific processes, rather than specific conditions, we can develop a mechanistic understanding that could enable an extrapolation beyond Earthly points of reference. For example, accessing deep ocean sediments enables us to study the dynamics of biological populations supported by extremely low fluxes of chemical energy (Hoehler and Jørgensen, 2013) such as may prevail on ice-covered ocean worlds, rather than the orders-of-magnitude greater energy that drives the distribution of biology in many other environments of our hydrosphere. Our exploration of ocean worlds will benefit most extensively when it leverages such process analogs in addition to the range of physical analogs that Earth offers.
The methods and technology that have been developed to explore Earth’s hydrosphere also serve as valuable design reference points for the exploration of worlds beyond Earth. For example, the development of technology for exploring subglacial lakes and under ice shelves currently informs, and provides crucial test opportunities for, high technological readiness level systems that can access and sample oceans in the outer solar system. The Whillans Ice Stream Subglacial Access Research Drilling (WISSARD) team that sampled West Antarctic lakes has pioneered rigorous, quantified control of forward contamination (Rack, 2016), which can now inform planetary protection approaches for ice-penetrating systems. The NASA Concepts for Ocean worlds Life Detection Technology (COLDTech) and Scientific Exploration Subsurface Access Mechanism for Europa (SESAME) programs have supported adaptation of terrestrial ice drilling and melt probe technologies to potential ocean world applications (Figure 2), development of communication technologies and navigation systems, and modeling of tectonic hazards and ice shell characteristics to reduce risks in flight hardware development. Similarly, NASA’s Planetary Science and Technology from Analog Research (PSTAR) program sponsored adaptation of deep-UV fluorescence and Raman-scattering methods to organic detection and characterization, as demonstrated on the Greenland ice sheet (Malaska et al., 2020). Beyond technology development, PSTAR also supports studies that develop mission “concept of operations” and explore specific observing strategies. As an example, the SUBSEA (Systematic Underwater Biogeochemical Science and Exploration Analog) PSTAR project brought together ocean and space scientists and operations experts in an effort to assess the best ways to conduct a remote science mission in an underwater ocean world environment.
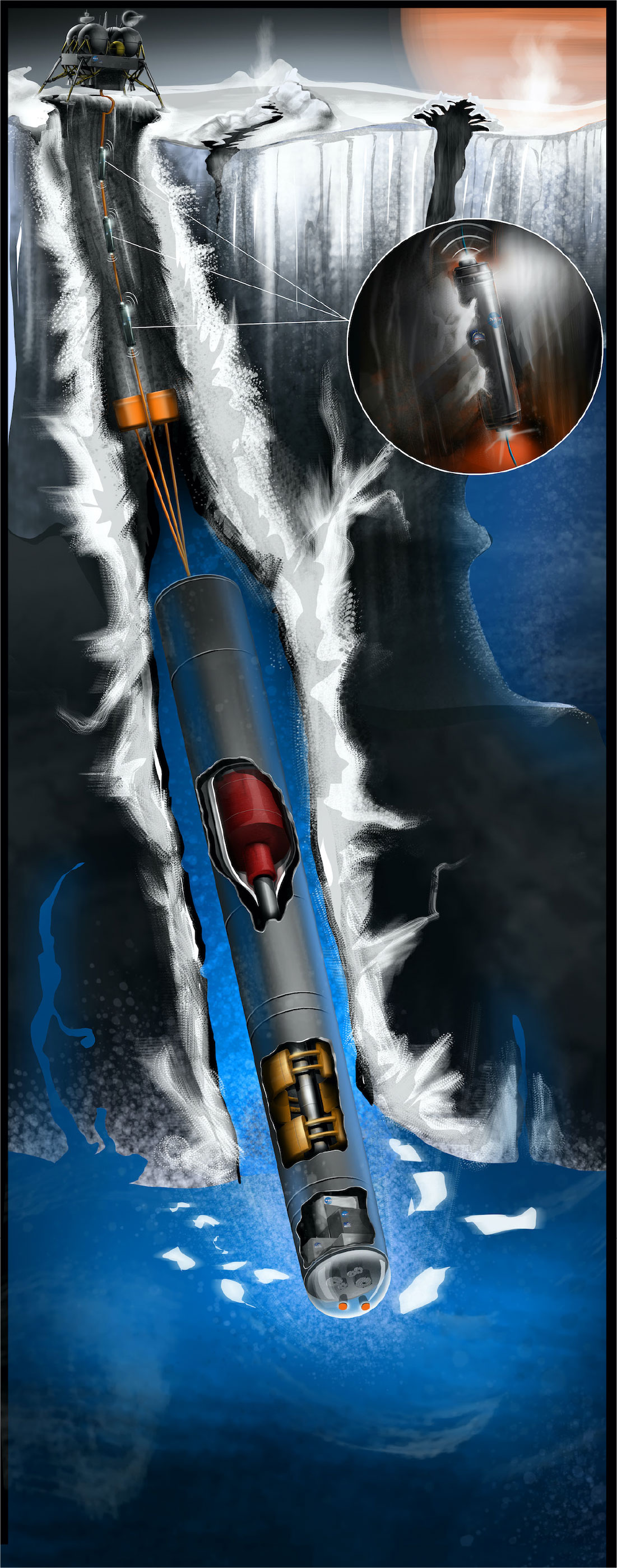
FIGURE 2. Artist’s concept for a cryobot probe to an ocean world (Oleson et al., 2019; image credit: Alexander Pawlusik). > High res figure
|
Summary
The ocean worlds of our solar system offer the intriguing potential to host extant life that, if present, would almost certainly have emerged independently of life on Earth. Advancement in exploration and measurement technologies leave us poised, as never before, to search for evidence of life on these worlds. Relative to Earth, the potential scarcity of resources implies that such evidence may be overall less abundant. However, we also know that the distribution of life and its products may vary by orders of magnitude across a land- or seascape, and thus result in local concentrations far above the global average. To optimize our chances of detecting evidence of life on ocean worlds therefore requires careful selection of targets, landing sites, and sampling and measurement strategies. This selection should be informed not only by existing observations of these worlds but also by an understanding of the processes that govern the distribution of life and its products across a land- or seascape. Our understanding of Earth’s hydrosphere, and the methods by which that understanding was built, offer an invaluable resource in this regard. We understand life on Earth as part of a broader system of processes that play out on a range of spatial and temporal scales. This system can be further characterized at scales ranging from microscopic to global, with methods that encompass and combine modeling, in situ analysis, and remote sensing. The results provide not just concrete points of reference, but an intellectual framework that can be applied to existing spacecraft observations in understanding where and how to search for evidence of life on the ocean worlds of our solar system.
Acknowledgments
TMH acknowledges support from NASA’s Planetary Science Division and from NASA grant 80NSSC19K1427. JSB was supported by NASA CAN8 80NSSC18K1301. KLC acknowledges support from NASA SESAME grant # 80NSSC19K0613. PAW’s contribution to the research was carried out at the Jet Propulsion Laboratory, California Institute of Technology, under a contract with the National Aeronautics and Space Administration (80NM0018D0004). DPW acknowledges support for this work from NASA grants NNX17AF68G and NNN12AA01C.