Introduction
Relative to their area, coastal ecosystems contribute disproportionately to global marine productivity (Field et al., 1998), with upwelling ecosystems accounting for the majority of that productivity (Chavez and Messié, 2009). Among the four major upwelling ecosystems, the California Current Large Marine Ecosystem (CCLME) is probably the most intensively studied (Checkley and Barth, 2009). However, knowledge of the patterns and dynamics of that portion of the CCLME closest to humans (i.e., the inner shelf ecosystem, defined as water depths generally less than 50 m) was limited and based mostly on local-scale, uncoordinated academic research. Here, we summarize the historical development of conceptual and methodological advances that spurred the 1999 creation of the Partnership for Interdisciplinary Studies of Coastal Oceans (PISCO), and then provide highlights of PISCO-driven advances in understanding the dynamics of the CCLME meta-ecosystem (meta-ecosystems are collectives of local ecosystems connected by flows of materials and energy; Loreau et al., 2003).
“In its first 20 years, PISCO has been a leader in scale-sensitive research that has yielded unprecedented insight into inner-shelf influences on pattern and process in shallow benthic communities.”
The Evolution of Experimental Ecology
In the mid-twentieth century, marine ecologists triggered an ecological revolution by demonstrating the power of manipulative field experiments to determine cause and effect (Connell, 1961; Paine, 1966). Previously, most researchers relied primarily on observations to explain community pattern and dynamics (Lubchenco and Real, 1991). By removing or excluding predators or competitors in controlled field experiments, Connell and Paine showed that species interactions influenced the distribution, abundance, and diversity of populations and communities. Although ecology remained dominated by observational approaches into the 1970s, by the 1980s, ecological research was increasingly experiment based, and species interactions commonly were identified as major determinants of ecological pattern. However, flaws in experimental approaches also were noted (Diamond, 1986; Inchausti, 1994; see review in Werner, 1998). For logistical reasons, most experiments were small scale (e.g., hundreds of square centimeters to a few square meters in area) and short term (e.g., days to a few years), thus limiting insights into the influence of larger-scale phenomena and limiting detection of time lags, natural temporal cycles, and population dynamics (Brown and Heske, 1990). Also, with exceptions (e.g., Dayton, 1971; Menge, 1976; Lubchenco and Menge, 1978), most experimental studies—whether marine, freshwater, or terrestrial—were done at single or a few sites, limiting their spatial generality. Because environmental gradients are universal in ecological systems (e.g., Whittaker, 1970), and marine studies indicated that interaction strength varied along such gradients (e.g., Dayton, 1971; Menge and Sutherland, 1976), incorporation of spatial variability into experimental designs was an important next step.
The Influence of Ocean Dynamics on Nearshore Ecological Patterns
Until ~1980, oceanic influences on ecological patterns in coastal marine ecosystems were underappreciated (e.g., Dayton and Tegner, 1984; Menge, 1992). Variable wave exposure as an important determinant of community structure and dynamics was the primary oceanic influence investigated (e.g., Lewis, 1964; Dayton, 1971; Menge, 1976), a focus that persists today (e.g., Bustamante and Branch, 1996; Taylor and Schiel, 2010; Bryson et al., 2014). However, with exceptions (Odum, 1980; Duggins et al., 1989, Witman et al., 1993), researchers largely neglected potential variation in the ocean’s role in delivering propagules, nutrients, and particulates (i.e., “ecological subsidies”; hereafter subsidy[ies]), thus implicitly assuming these inputs were relatively homogeneous. The 1980s saw a paradigm shift when researchers began investigating recruitment effects on intertidal communities (Underwood and Denley, 1984; Gaines and Roughgarden, 1985), and including recruitment in models of community dynamics (Menge and Sutherland, 1987). These advances inspired a 1987 US National Science Foundation-funded workshop (Eckman et al., 1989) that proposed two new directions in marine research: (1) expansion of spatial and temporal scales, and (2) inclusion of ocean-driven subsidies.
Research in the 1990s demonstrated the influence of subsidies on community and population structures (e.g., Menge, 1992; Bustamante et al., 1995; Polis and Hurd, 1996; Dayton et al., 1999). For example, filter-feeding basal species growth varied with inputs of phytoplankton (Menge, 1992) and kelp detritus, algal productivity varied with upwelling (Bustamante et al., 1995), and terrestrial food web dynamics varied with marine-derived carcasses and wrack among different-sized islands (Polis and Hurd, 1996).
Looking Toward a “Grand Unified Theory of Ecology”
Ecology is theory-rich, but because ecological systems involve interactions among living, evolving, variable, diverse biota and many equally variable environmental factors, understanding ecosystem dynamics remains a work in progress. In fact, the likelihood of achieving a “grand unified theory of ecology” remains controversial (e.g., Lawton, 1999; Simberloff, 2004; Ricklefs, 2008; Brooker et al., 2009).
Nonetheless, community theory has advanced. Highlights include establishing “top-down” (Hairston et al., 1960) and “trophic cascades” concepts (Paine, 1980; Carpenter et al., 1985), where predators indirectly determine plant community structure by controlling herbivore abundance. Years of research showed strong top-down dynamics in natural systems (e.g., Terborgh and Estes, 2010). Yet, studies also revealed how bottom-up control, via spatial variation in subsidies, could underlie variation in trophic structure (i.e., number of trophic levels; Oksanen et al., 1981; Fretwell, 1987) or affect the importance of predation (Power et al., 1996; Borer et al., 2005). Top-down influences often counter negative effects of competition, which is nontrophic, with important consequences for community structure (e.g., Paine 1966). Alternatively, nontrophic facilitative (positive) interactions can be important, particularly in moderating effects of stress (Bertness and Hacker, 1994; He et al., 2013). Positive interactions may rival top-down effects in influencing species abundance and richness, at least among foundation species (Thomsen et al., 2018).
Plant ecologists were among the first to document that communities varied in structure along environmental gradients (Whittaker, 1970). Building on the top-down/bottom-up perspective, ecologists identified environmental stress as a determinant of variation in species interactions and of their role in structuring communities (Connell, 1975; Menge and Sutherland, 1976; Grime, 1977). The identification of propagule input rates as drivers of species abundances led to the incorporation of recruitment density gradients—along which competition could be strong (high recruitment) or weak (low recruitment)—into the expanding conceptual model framework (Menge and Sutherland, 1987). The later incorporation of facilitation yielded the present “environmental stress model” framework (Bruno et al., 2003; Silliman and He, 2018). In synthesizing these ideas, it became clear that conducting field experiments and observations across large spatial and long temporal scales would be necessary in order to approach a “grand unified theory of ecology.”
PISCO: Purpose and Vision
A main focus of PISCO was on the biogeography of ecological processes. Our overarching question was: How do major elements of the CCLME function to produce patterns of species distribution, abundance, and diversity? Because determination of climate change impacts requires lengthy data sets, we planned for PISCO to last decades. Because the ocean varies across scales of tens to thousands of kilometers, we envisioned conducting identically designed and executed studies at multiple sites along the North American west coast. Finally, because multiple biotic and abiotic processes interact to determine ecological patterns, we aimed to quantify patterns, evaluate the processes driving those patterns, and investigate the factors underpinning species’ responses to environmental processes.
Coastal Ocean Context: A “Black Box”
Understanding the role of inner-shelf ocean processes in shaping nearshore ecosystems was and remains a key PISCO goal. Although earlier technology demonstrated variable oceanic conditions, satellite images of sea surface temperature (SST) revealed unexpected texture at multiple scales (Figure 1). Further, features such as upwelling centers appeared to be anchored to coastal features such as headlands, bottom topography, and coastal angle. SST maps revealed local-to-regional upwelling variation that guided the design of the PISCO study site array (see Figure 1 in Menge et al., 2019, in this issue).
Despite these insights, however, satellite imagery could not resolve “inner-shelf” oceanic features. Moreover, shallow inner-shelf depths precluded sampling by large oceanographic vessels. Because the inner shelf was an oceanographic and ecologically data-poor “black box,” establishment of instrumented inner-shelf mooring networks in Oregon and central and southern California was a top priority (Cudaback et al., 2005; Kirincich et al., 2005). In addition to temperature, salinity, and current sensors, moored instrumentation included chlorophyll-a sensors, and, later, oxygen and pH sensors (see Chan et al., 2019, in this issue). These arrays helped provide the first comprehensive look at inner-shelf coastal oceanography along the CCLME.
To capture interactions between the inner shelf ocean, intertidal, and subtidal benthic ecosystems, we used a nested research design. Local-scale rocky-reefs or nearby kelp beds, termed “sites” (with scales of tens to hundreds of meters), were basic spatial units. Successively larger scales were replicate sites of similar biological structure nested within coastal sectors, or “capes” (kilometers to tens of kilometers), replicate capes nested within “subregions” (tens to hundreds of kilometers; i.e., with similar oceanic conditions), and subregions nested within “regions” (scales of hundreds to thousands of kilometers).
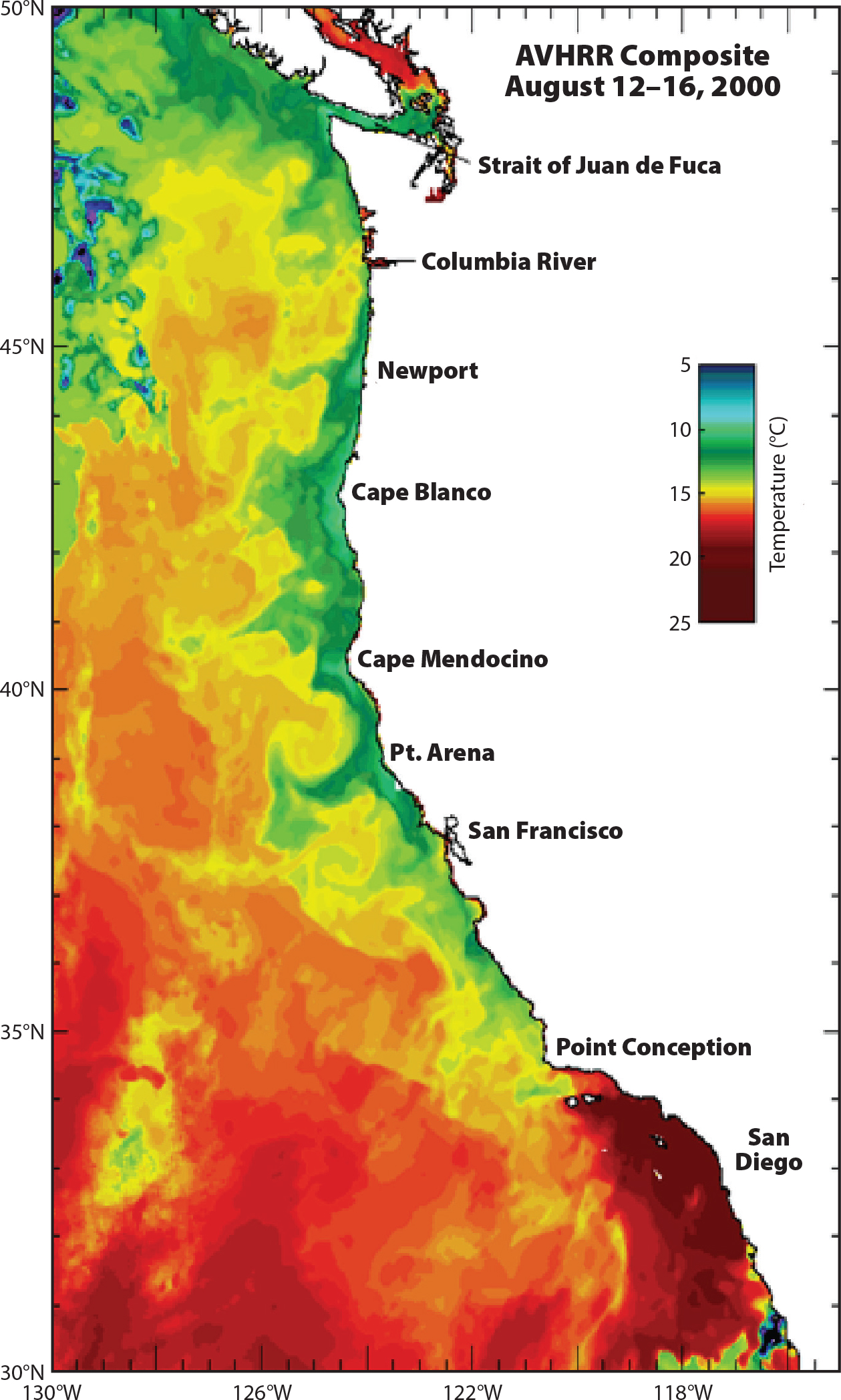
Figure 1. Sea surface temperature along the California Current System. > High res figure
|
Ecological Subsidies
Understanding the ocean’s role in structuring coastal ecosystems required quantification of subsidies connecting inner shelf-kelp bed-intertidal communities. Based on prior methods (e.g., Farrell et al., 1991; Menge, 1992) and pre-PISCO data sets (Connolly et al., 2001) together with new method development (Ammann, 2004; White et al., 2019, in this issue), we established a coast-wide program of frequent sampling (biweekly to monthly).
Major Advances
Although PISCO research over the past 20 years focused on the CCLME and advanced understanding of its dynamics, our studies contributed more generally by providing (1) conceptual advances relevant to other LMEs, and (2) broader ecological conceptual advances applicable to marine and nonmarine ecosystems alike. The following examples illustrate these advances.
Widespread Application of Nested Designs
A key PISCO advance was application of the comparative-experimental approach (CEA) across large spatial scales (i.e., using geographically nested designs to understand large-scale dynamics at the meta-ecosystem scale). Understanding meta-ecosystems based on observations is challenging because conducting process studies, particularly manipulations, is largely unfeasible at the large spatial scales spanned by meta-ecosystems. Further, site-scale removals or additions of species are logistically challenging and ethically questionable. The CEA offers a feasible alternative. As pioneered by Dayton (1971), the CEA combines identically designed, executed, and replicated small-scale manipulations at multiple sites along environmental gradients typical of oceanic variability (e.g., disturbance, upwelling, temperature, salinity, pH, currents). Besides Dayton’s (1971) seminal work, CEA studies have been done in New England (Menge, 1976; Lubchenco and Menge, 1978; Bryson et al., 2014), Europe (Coleman et al., 2006), South Africa (Bustamante et al., 1995), Chile (Navarrete et al., 2005), New Zealand (Menge et al., 2003), the Galápagos (Witman et al., 2010), and Oregon (Menge et al., 1997).
Consumer pressure (consumptive and nonconsumptive predation, herbivory) can be a powerful structuring force (e.g., Shurin et al., 2002; Terborgh and Estes, 2010; Kimbro et al., 2017) but is not universally strong (Menge, 1976; Lubchenco and Menge, 1978; Arnott and Vanni, 1993; Freestone et al., 2011; Bryson et al. 2014; Kimbro et al., 2017). As summarized earlier, understanding variation in ecological processes requires investigations along environmental gradients. One such gradient, subsidies, can be an important factor driving trophic structure, and thus consumer pressure (Menge and Sutherland, 1976, 1987; Fretwell, 1987; Oksanen et al., 1981; Carpenter et al., 1985; Menge et al., 1997; Kimbro et al., 2019).
Models predicted that upwelling variability would drive recruitment inputs that would underlie variation in top-down effects (Roughgarden et al., 1988; Connolly and Roughgarden, 1999). This scenario (the “recruit-adult” model) assumed passive transport of larvae and phytoplankton driven offshore during upwelling and onshore during downwelling. PISCO studies were consistent with the latter predictions (Figure 2), but the top-down response remained untested. To gain insight into top-down and bottom-up variability, we asked: How does consumer pressure vary in relation to subsidies across large spatial scales? Using simple methods, we quantified variation in sea star predation rate (Pisaster ochraceus) on mussel prey (Mytilus californianus) along a coastal gradient of oceanic conditions and subsidies (Menge et al., 2004). Regressions showed that mussel recruitment (Figure 2a; adj. R2 = 0.678) and phytoplankton abundance (adj. R2 = 0.315) both varied inversely with upwelling. However, our experiments did not support the recruit-adult model. Predation rates were unrelated to subsidies (phytoplankton p = 0.39, mussel recruitment p = 0.3), instead increasing with sea star density (adj. R2 = 0.46). In categorical analyses, however, predation rate was at least three times greater when a site had high recruitment and high phytoplankton (Menge et al., 2004). We concluded that in the CCLME, predation rate was multifactorial, driven by ecological subsidies (especially in the north) and factors underlying the abundance of P. ochraceus (e.g., alternative prey, sea star recruitment, and sea star longevity).
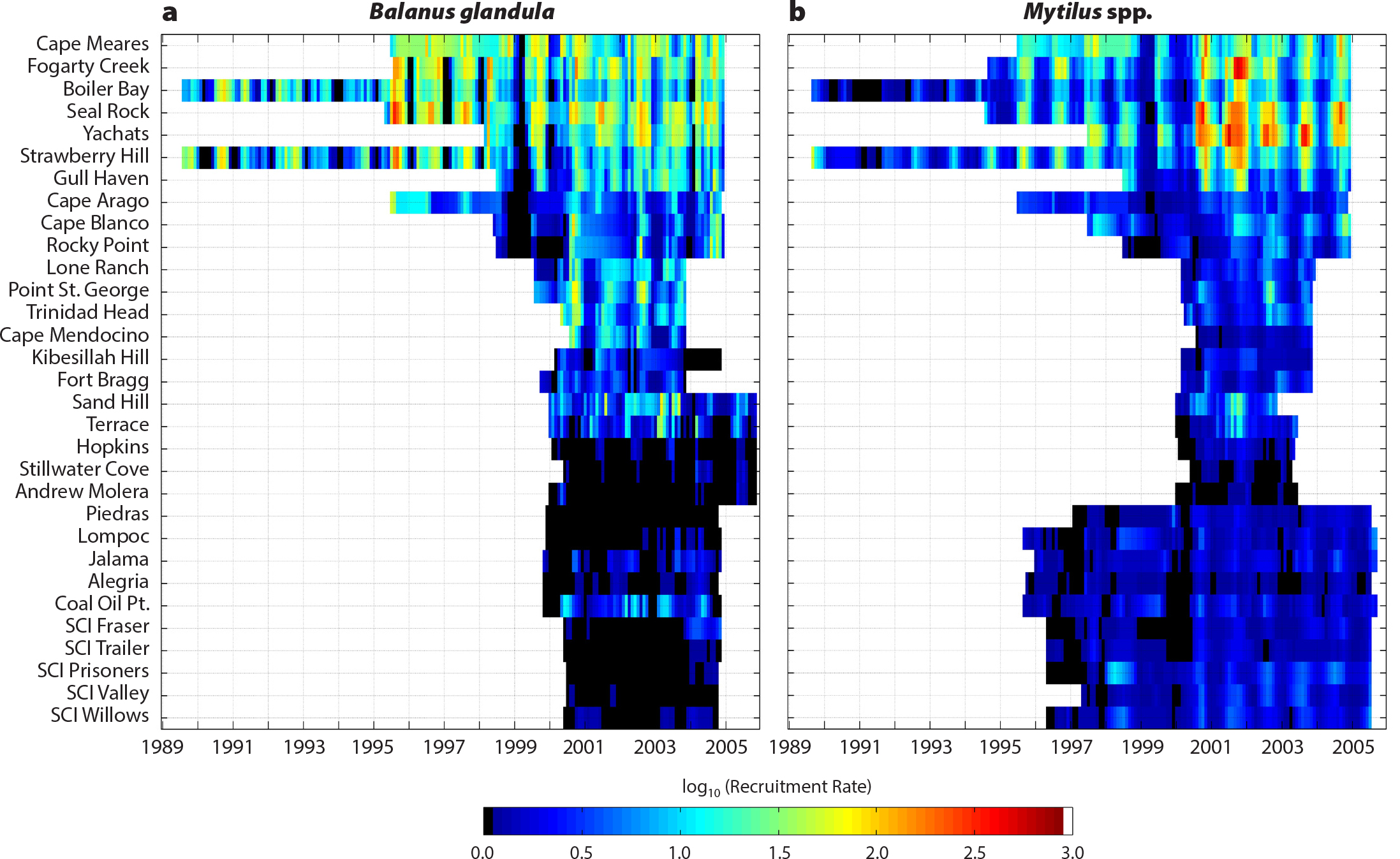
Figure 2. Monthly larval recruitment rate time series from January 1997 to December 2004 for (a) Balanus glandula, and (b) Mytilus spp. at the 31 study sites, arranged from north to south. Larval recruitment rates in central Oregon (Fogarty Creek to Strawberry Hill) are more than five orders of magnitude greater than in central California (Hopkins to Piedras), but increase again toward Point Conception (Jalama). Note the clear annual cycles across the region, the marked decline in recruitment south of Cape Arago, and the near-absence of recruits south of Monterey Bay (Hopkins). Recruitment rate was the number of recruits per day per larval collector, transformed prior to analyses. Black indicates zero recruitment, and white indicates no data. From Broitman et al. (2008). > High res figure
|
Latitudinal Subsidy Gradients
Geographic subsidy quantification revealed dramatic nonlinear barnacle and mussel recruitment gradients. Mussel and Balanus glandula (barnacle) recruitment was high in central Oregon, dropped abruptly at Cape Arago, decreased further between Monterey Bay and Point Conception, and increased slightly southward of Point Conception (Broitman et al., 2008; Figure 2).
Phytoplankton (proxied by chlorophyll-a) varied similarly, with higher levels northward than southward (Barth et al., 2007; Hickey and Banas, 2008). Phytoplankton abundance and recruitment were strongly associated with continental shelf width (Hickey and Banas, 2008; Menge et al., 2015). Wide shelves have higher retention and more sluggish currents (Kirincich et al., 2005; Hickey and Banas, 2008), suggesting an important effect of flow-topography interactions on subsidies (Menge et al., 2015). In contrast to phytoplankton, nutrients (NO3– and NO2–) varied primarily through time in concert with upwelling events but only weakly with latitude (Barth et al., 2007; Hickey and Banas, 2008).
Mechanisms Linking Local, Regional, and Coast-Wide Ecosystems
Are inner-shelf processes causally linked to community dynamics? As noted above, the recruit-adult model hypothesized that variable cross-shelf upwelling currents determined geographic differences in recruitment rates, thereby altering community structure (Roughgarden et al. 1988; Connolly and Roughgarden, 1999; Botsford et al., 2006). The model predicted low recruitment with persistent upwelling (because of continual offshore larval transport) or persistent downwelling (because of low larval survival due to limited food availability).
Do upwelling regimes really underlie patterns of recruitment? The data in Figure 2 are consistent with this idea, as are literature examples (e.g., Menge et al., 2003; Caselle et al., 2010; Lathlean et al., 2019). However, research revealed that larvae are not passive particles. Rather, using vertical migration, some larval species can move vertically into water layers that keep them within a few kilometers of the shore (Morgan et al., 2009), indicating that recruitment dynamics are more complex than simple demographic linkages would suggest.
An alternative model, the “surf-zone hydrodynamics” hypothesis, suggests that upwelling currents do not affect onshore recruitment (e.g., Shanks et al., 2017). Instead, this hypothesis argues that the surf zone imposes a semi-permeable barrier (e.g., Rilov et al., 2008) that modulates successful larval transport from ocean to shore (e.g., Morgan et al., 2016; Shanks et al., 2017). That is, successful recruitment depends on onshore movement of water by wave action, internal waves (e.g., Pineda, 1999), tidal change, and shore topography. Waves cross broad “dissipative” beaches relatively slowly, depositing larvae as they go, whereas waves crossing steep “reflective” beaches rebound seaward, preventing larval settlement (terminology of McLachlan, 1990). Shanks et al. (2017) suggested that this mechanism applies to barnacle recruitment on rocky shores. However, independent analyses found no relationship between barnacle (or mussel) recruitment and surf zone width (Menge and Menge, 2019).
The larval vertical migration hypothesis (Morgan et al., 2016) suggests that this mechanism keeps larvae within a few kilometers of shore. Thus, because larvae still must travel several kilometers shoreward to reach the surf zone and intertidal, cross-shelf transport seems required. This suggestion was borne out by three studies. In South Africa (Benguela Current System), shoreward larval transport depended on the upwelling regime. As larvae approached shore, wave action, tidal change, and sea breezes explained additional variance in barnacle and mussel settlement (Pfaff et al., 2015). In the CCLME, barnacle, mussel, and fish recruitment depended on the proximity of ocean fronts to the shore (Woodson et al., 2012). In southern California, fish recruitment depended on both large-scale and small-scale ocean factors (Caselle et al., 2010). Large-scale factors were larval production and early survival, which are dependent on basin- and regional-scale processes, while small-scale factors were local-scale processes affecting larval delivery to nearshore habitat. Thus, successful recruitment depends on a complex series of ocean and biotic processes (e.g., Pineda, 2000). A recent synthesis suggests how these subsidy delivery mechanisms link together to replenish benthic populations and highlights areas where further understanding is needed (Figure 3; Menge and Menge, 2019).
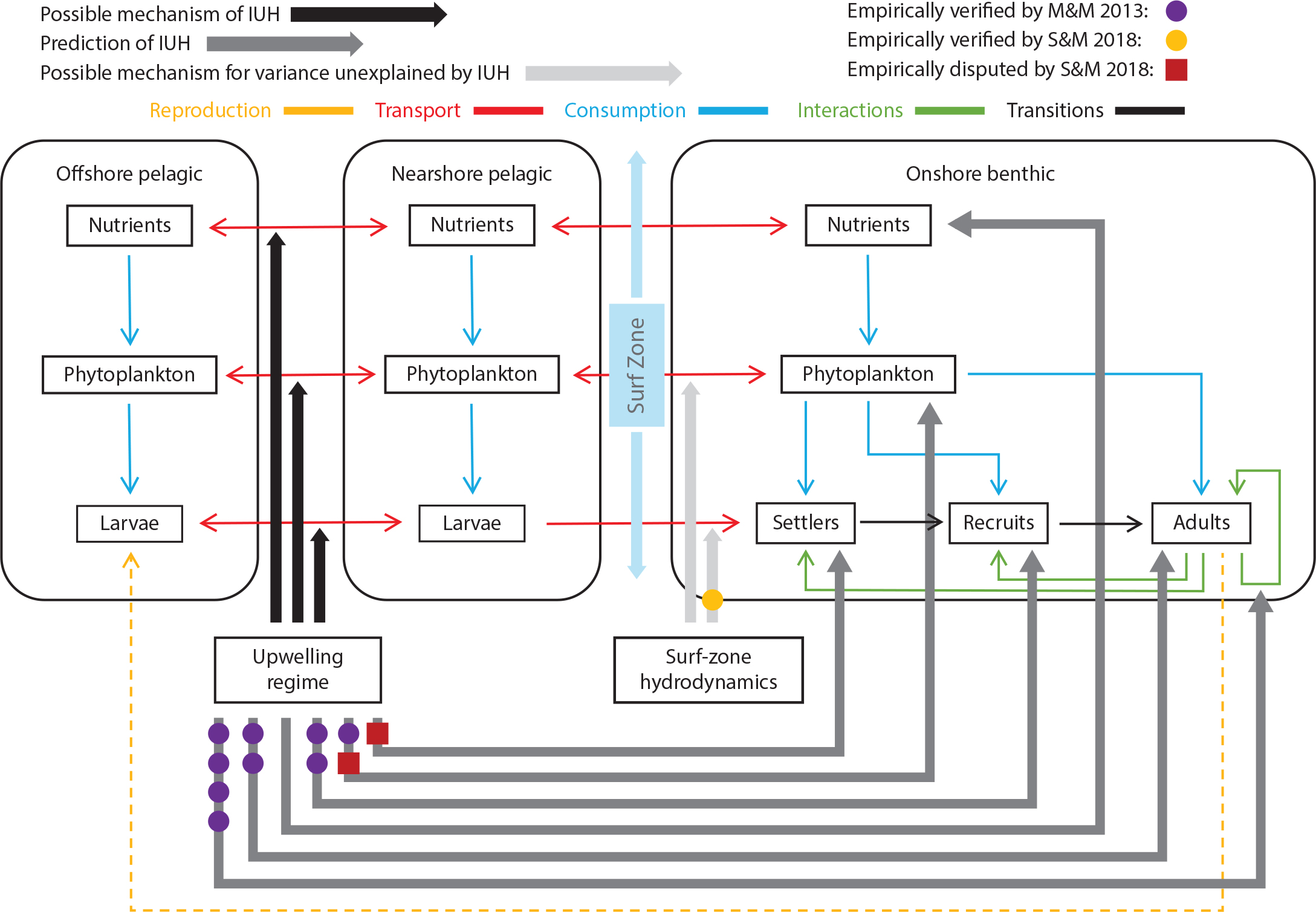
Figure 3. Conceptual model of benthic-pelagic coupling. Codes for symbols and arrows are shown at top of diagram. Boxes represent offshore environments (i.e., ±5 km from shore) where larvae reside during development, nearshore environments (500–1,000 m from shore) where oceanic/behavioral processes stage them for transport through the surf zone (i.e., the space between nearshore and onshore), and onshore environments where settlement, recruitment, and growth to adulthood occur. Predictions that were supported by Menge and Menge (2013) data are marked with purple dots. Mechanisms supported by Shanks et al. (2017) and Shanks and Morgan (2018) for sandy shores and sandy shores with embedded rocks are marked with a yellow dot. Controversial mechanisms (see Menge and Menge, 2019) are marked with red boxes. Modified from Menge and Menge (2019). > High res figure
|
Spatial Marine Ecology: Integration of Oceanic and Benthic Linkages into a Meta-Ecosystem Framework
PISCO is strongly place-based and focused on gaining a spatial perspective. We also aim to generate and transfer knowledge to other geographic regions. For example, recent syntheses test meta-ecosystem theory and thereby provide evidence-based frameworks for integrated understanding of coastal ecosystem patterns and dynamics (Menge and Menge, 2013; Menge et al., 2015; Hacker et al., 2019). A major challenge of spatial ecology is understanding the dependence of community structure on local- versus larger-scale processes. For benthic communities, alternative hypotheses include local structure (1) is determined entirely by oceanic processes, (2) varies jointly with oceanic processes and local-scale processes, or (3) is idiosyncratic, with little influence from oceanic processes (Figure 1 in Menge et al., 2015).
We tested these ideas in the northern CCLME (Bodega Marine Lab to north-central Oregon; Menge et al., 2015). We quantified intertidal community structure and subsidies and related these to oceanic and environmental measurements. Of the community variance explained by spatial and temporal factors, approximately 52% of was explained by large-scale spatial variation, 27% was explained by local-scale variation, and 18% was explained by time (Figure 4). At the northern CCLME-scale, upwelling intensity, nutrients, and canopy cover varied negatively, and phytoplankton, recruitment, and sessile invertebrate abundance varied positively with shelf width (Figure 5). Nutrient levels and invertebrate abundance increased, and recruitment decreased with upwelling. Sessile invertebrate abundance increased and algal turf cover decreased with increasing phytoplankton, while invertebrate-predator abundance increased with invertebrate-prey abundance. Water temperature was independent of any of the potential driving factors, and negatively associated with herbivore abundance, while herbivore abundance had only a weak positive association with nutrients. Similar proportions of variability explained ecosystem dynamics (Hacker et al., 2019). That is, of total community variance explained by CEA-based experiments, large-scale processes explained 40%–49% while local-scale interactions explained 19%–39%.
In the southern CCLME, the cooler, equatorward-flowing California Current meets the warmer, northwestward-flowing Southern California Counter Current at Point Conception. Spatial patterns in intertidal and kelp forest community similarity correspond strongly with the thermal structure of this region, creating distinct “bioregions” (Blanchette et al., 2008; Claisse et al., 2018). However, local environmental variables such as temperature also correspond with patterns of ocean-mediated dispersal because both are strongly influenced by currents. Sorting the relative importance of local thermal environments from regional dispersal patterns for community structure is challenging. To separate these effects, we partitioned the statistical contribution of ocean-mediated dispersal from that of local thermal structure on spatial community similarity (Watson et al., 2011). Using ocean circulation modeling, we created a novel set of metrics—oceanographic “distance” (average dispersal time between sites) and “asymmetry” (difference between outgoing and incoming dispersal times at a site). These region-scale metrics corresponded more closely with intertidal and subtidal community similarity than did local-scale thermal structure of the domain, suggesting that ocean-mediated dispersal exerted the dominant effect on spatial patterns of nearshore community similarity.
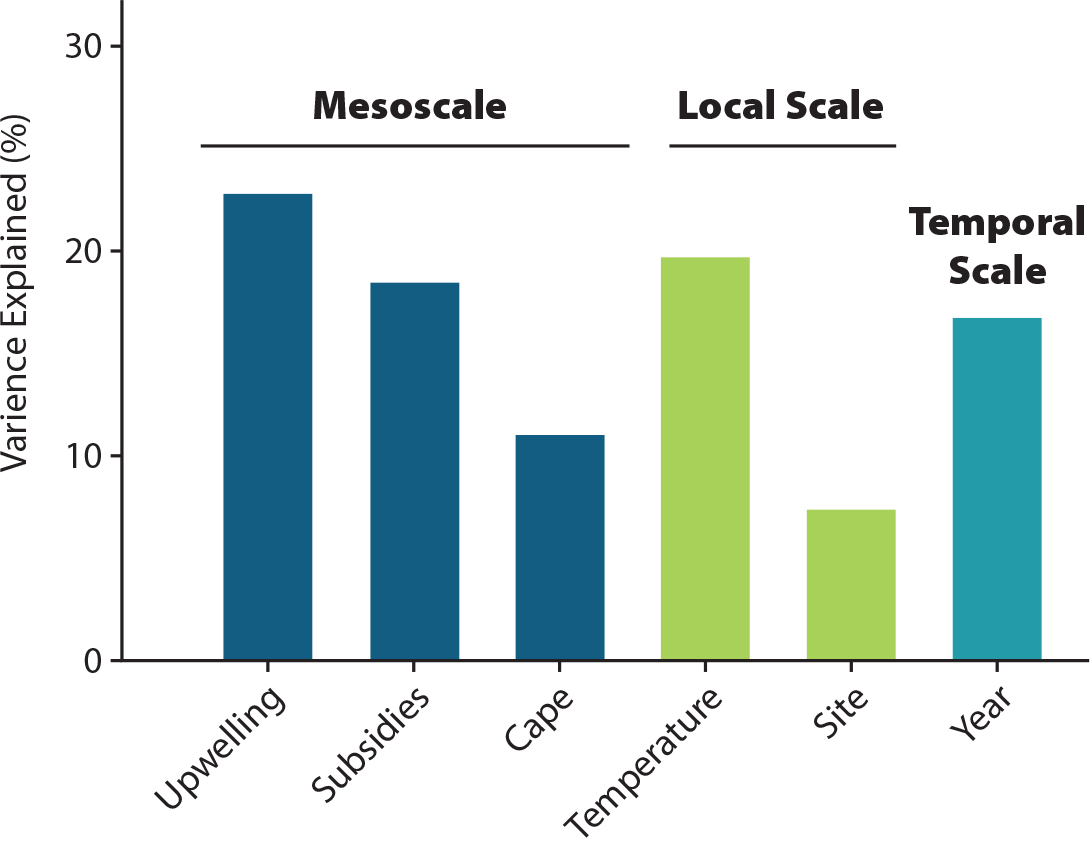
Figure 4. Percentage of variance in community structure explained by environmental factors, ecological subsidies, space, and time, analyzed by PERMANOVA and arranged by scale. From Menge et al. (2015). > High res figure
|
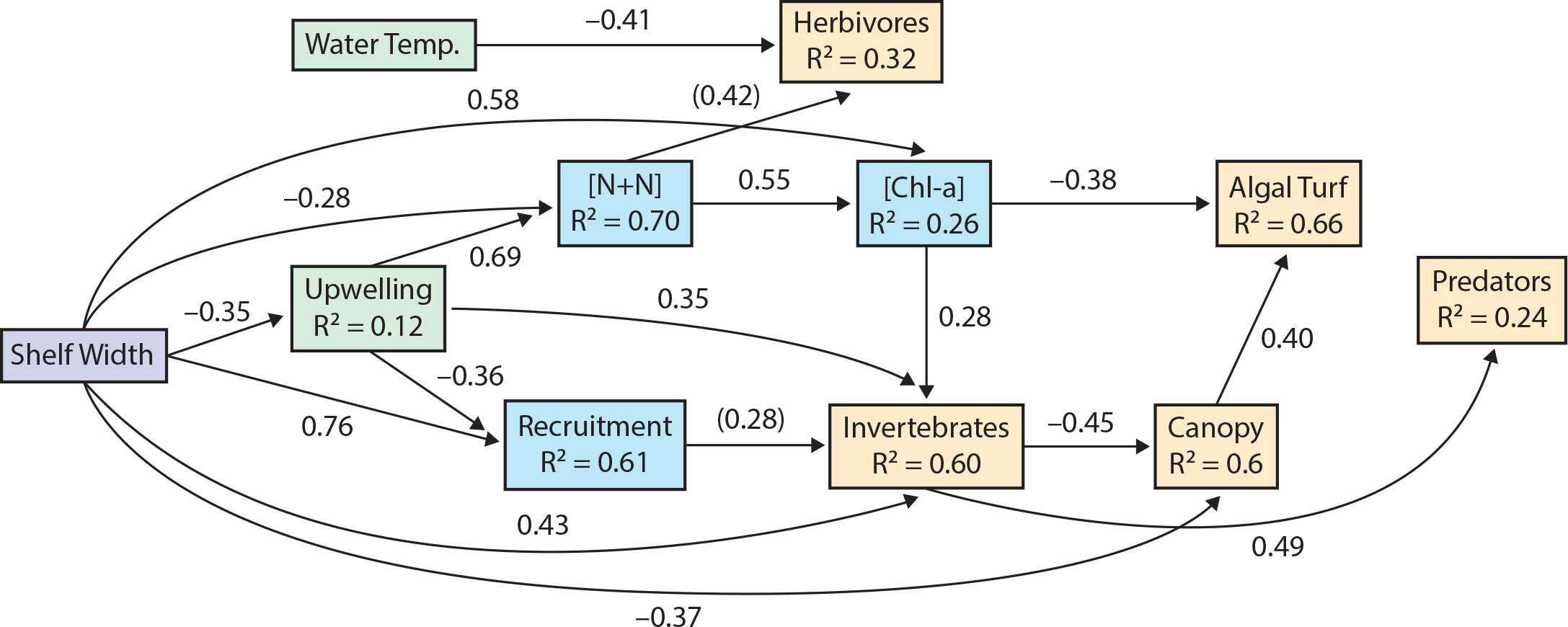
Figure 5. Path analysis showing the hierarchical flow of effects from larger to more local scales (indicated by shading from shelf width (lavender), to upwelling (light green), to ecological subsidies (light blue), to components of local community structure (light yellow). Numbers by arrows are standardized path coefficients, which represent the predicted response in units of standard deviations. Coefficients significant at p <0.1 are in parentheses; other coefficients are significant at p <0.05. R2 values represent the amount of variation in response variables explained by all independent variables pointing to them. Surprises were a lack of links from upwelling or shelf width to water temperature, and N+N to algal turf and canopy. From Menge et al. (2015). > High res figure
|
Geographic-Scale Ecology: The Intermittent Upwelling Hypothesis
Expanding the CEA to an inter-hemispheric scale, Menge and Menge (2013) proposed that upwelling regimes are a major global determinant of intertidal community structure. They hypothesized that variation in structure was driven by the relative frequency of alternation between upwelling and relaxation/downwelling. The proposed mechanism is that upwelling supports high productivity in surface waters, while periodic relaxations in upwelling halt cross-shelf transport, keeping productive waters and planktonic propagules over the inner shelf. This “intermittent upwelling hypothesis” (IUH) suggests that rates of ecological processes (predation, competition, subsidies, growth) are high with intermittent upwelling (i.e., when switches between upwelling and relaxation/downwelling occur relatively frequently, roughly weekly to biweekly). In turn, all rates are predicted to be low with either persistent upwelling or persistent downwelling, that is, they occur roughly continuously, with only brief switches to the opposite condition.
Menge and Menge (2013) combined PISCO CEA results with their parallel research done in New Zealand to test this hypothesis. Factors investigated included phytoplankton abundance (closely correlated with phytoplankton productivity; Menge, 2000) and rates of recruitment, mussel growth, barnacle colonization, predation, and competition. In all cases, the relationship between process rates and upwelling regime were unimodal as predicted (Menge and Menge, 2013), and subsidies were strongly correlated with community dynamics (Figure 6).
Importantly, complete tests of the IUH require quantification of the full range of upwelling regimes, from persistently downwelled through intermittently upwelled to persistently upwelled regions. However, more geographically limited partial tests are also possible. For example, Lathlean et al. (2019) tested the persistent-downwelling to intermittent-upwelling half of the IUH. They quantified barnacle recruitment in intermittently upwelled (southeastern South Africa) and persistently downwelled (southeastern Australia) regions. As predicted, barnacle recruitment was higher in intermittently upwelled locations. A potentially similar result was obtained in a study in the Galápagos (Witman et al., 2010), where predation and ecological subsidies increased with increasing upwelling, with no sign of unimodality. However, it was unclear if the upwelling regime in this study spanned the full upwelling regime assumed by the IUH.
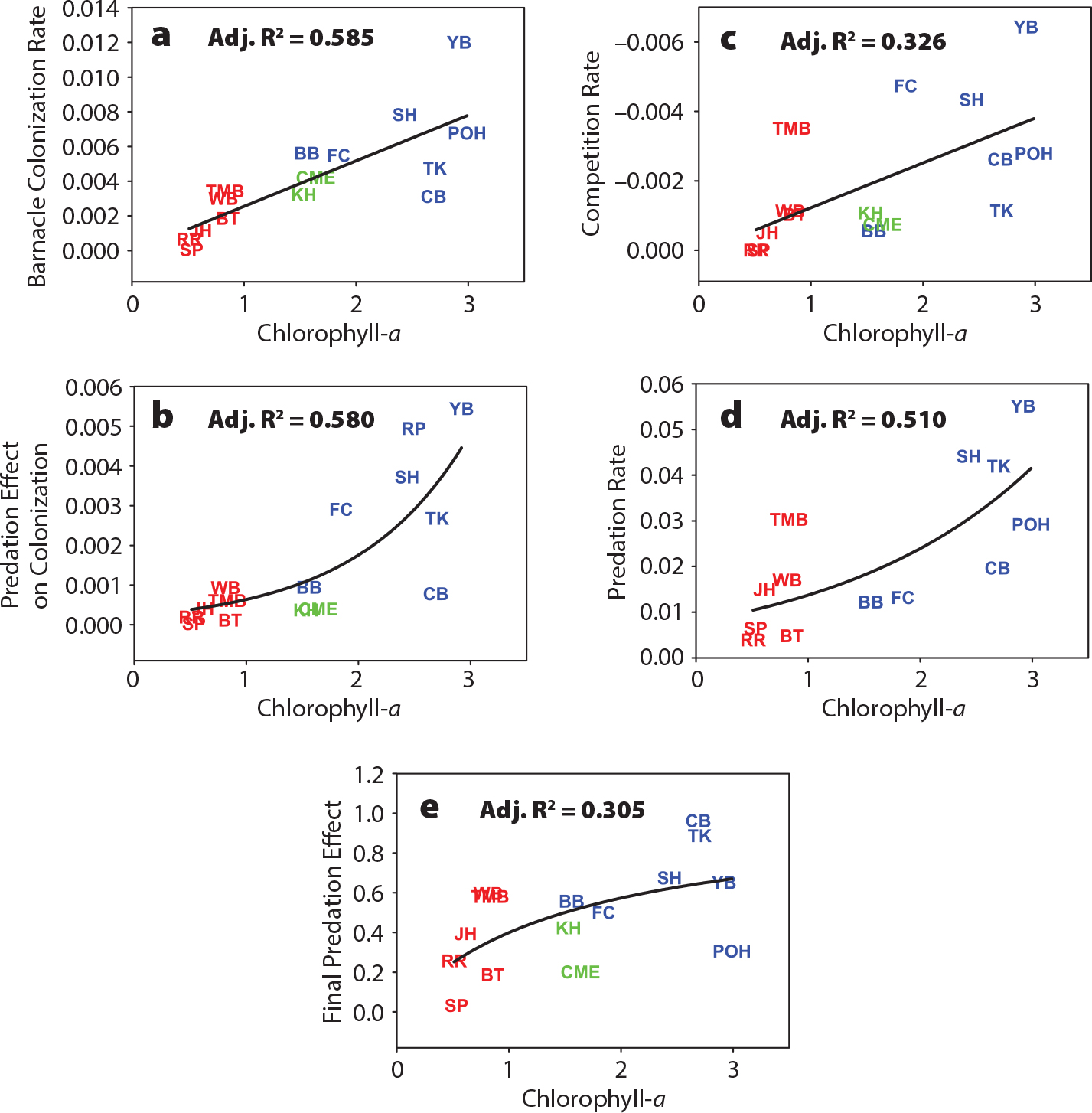
Figure 6. Correlations between the natural logarithm of chlorophyll-a and (a) barnacle colonization rate, (b) effect of predation on prey colonization rate, (c) space competition rate (barnacles vs mussels), (d) predation rate (sea stars on mussels), and (e) effect of predation on final prey abundance in exclusion experiments. Symbols are for Oregon (blue), California (green), and New Zealand (red) study sites. Adj. = adjusted. From Menge and Menge (2013). > High res figure
|
Conclusions
In its first 20 years, PISCO has been a leader in scale-sensitive research that has yielded unprecedented insight into inner-shelf influences on pattern and process in shallow benthic communities. Advances were made across local to large spatial scales that clarified the meta-ecosystem dynamics of the CCLME, knowledge that suggests testable hypotheses applicable to other coastal LMEs and to nonmarine meta-ecosystems. The research sharpened understanding of key ecological concepts such as top-down/bottom-up impacts, the role of ecological subsidies, and how these are driven along environmental gradients. We pioneered widespread application of the CEA, thereby enabling insights into geographic patterns of meta-ecosystem dynamics and highlighting an approach that can be used in any system. Similar groups have focused on ecosystem dynamics in kelp beds (the Kelp Ecosystem Ecology Network, KEEN, http://www.kelpecosystems.org/) and seagrass communities (the Zostera Experimental Network, Zen, http://zenscience.org/), and we urge expansion of this approach to other systems to provide society with crucial understanding of ecosystems globally.
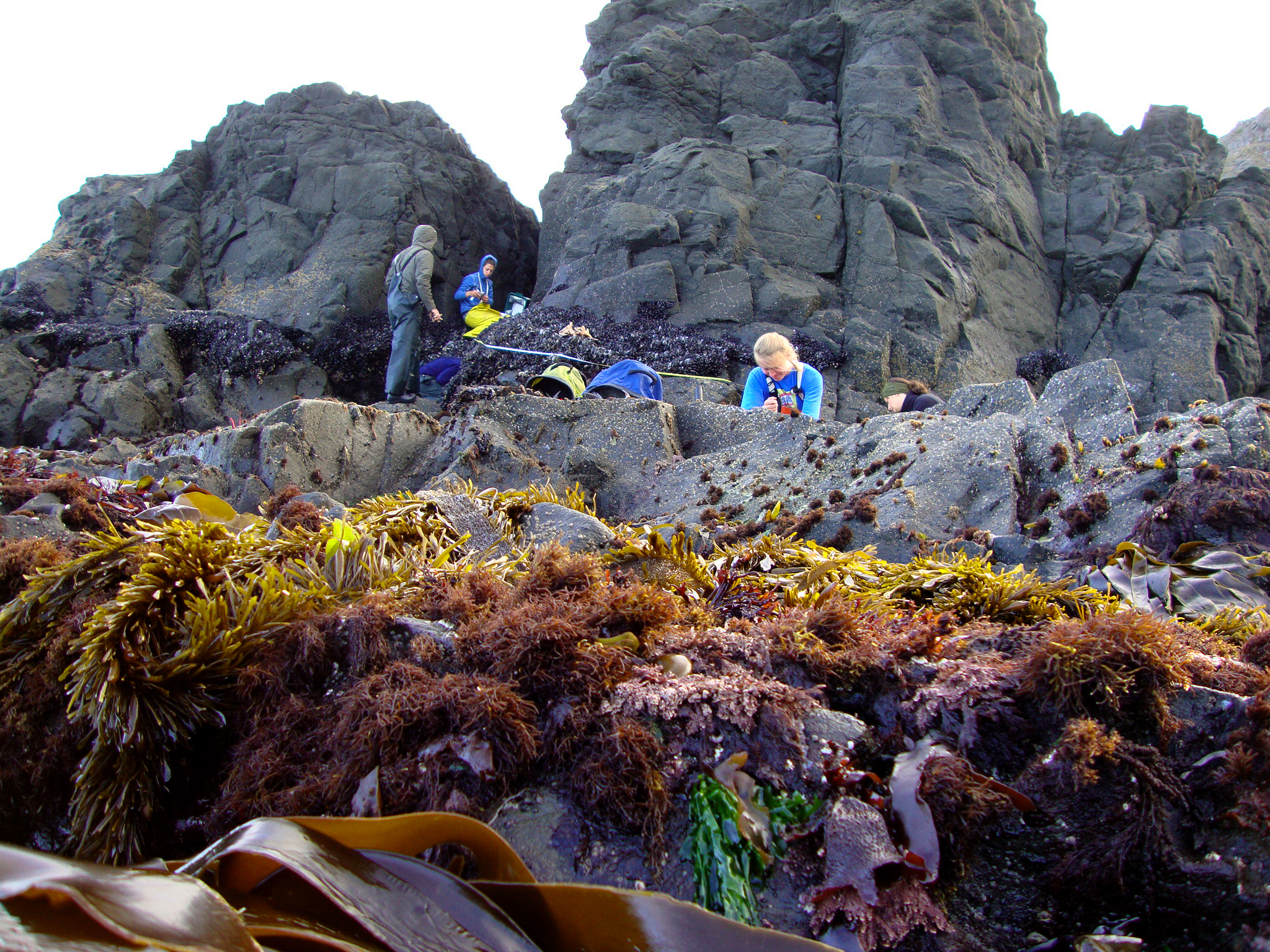
Researchers conducting surveys and monitoring experiments at Cape Blanco, Oregon. Foreground seaweeds include brown kelps, red bladed and foliose algae, pink coralline algae, and green algae. Above the algae is a mostly bare rock zone and a mussel zone. Photo credit: Heather Fulton-Bennett. > High res figure
|
Acknowledgments
We thank the many research assistants, graduate students, and postdoctoral scholars who have contributed so much to PISCO. Our research was supported by the David and Lucile Packard, Gordon and Betty Moore, and Andrew W. Mellon Foundations, and grants from the National Science Foundation. This is PISCO publication number 491.