Introduction
Several studies have been published to date about the impact of deep-ocean warming on acoustic propagation, in particular, in the Arctic Ocean (see references in Arctic section below). However, for a number of reasons, the impact of ocean warming on acoustic propagation over continental shelf and slope regions has received far less attention. First, the physical oceanographic processes acting in shallow regions, such as strong internal tides and internal waves, tend to be more complex. Second, bottom attenuation plays an important role in acoustic propagation, and in many regions, sub-bottom geo-acoustic properties are not well known. Third, there are many frontal zones present in most shelf/slope regions, and the significant sound speed differences that occur across frontal zones lead to complex acoustic energy redistribution as the sound propagates through the frontal gradient. Thus, there are many processes, ranging over widely varying spatial and temporal scales, to consider before we can understand how climate change affects shallow-water acoustic propagation. Despite such difficulties, reasonable estimates of changes over time and space are feasible.
In this paper, we consider two important and representative regions where warming of the continental shelf and slope is pronounced: the northeastern United States and the western Arctic. The reasons for such a study are numerous, and like the majority of issues associated with climate change, the effects are not good news for humanity. They can be broken roughly into military, civilian, and scientific reasons for discussion purposes.
The military, in particular the United States Navy, has long been concerned with climate change effects (e.g., National Research Council, 2010). Navies rely heavily upon acoustic sensors and systems, and their performance in various regions will significantly change. The signals, noise, and propagation conditions that are key components of the sonar equation all can, and likely will, change appreciably. Perhaps the clearest example of this to date is in the Arctic, where ocean warming has reduced the surface ice pack (an acoustic loss mechanism) and, in the western Arctic, has also created a subsurface sound duct that channels sound extremely well. As another example, in mid-latitudes, changing propagation conditions due to greater spatial variability in the position of fronts as well as large changes in frontal gradients will change Navy acoustic prediction maps for detection and localization.
On the civilian side, perhaps the most significant changes resulting from ocean warming will be shifts in the composition of the ambient noise field, which affects marine life and fisheries. The warming of ocean waters has pushed warm water species further north, changing the acoustic “soundscape” at a given location (Miksis-Olds et al., 2018). Another factor affecting marine life is changing shipping routes, which will introduce more noise in places where it was lower previously. This is already happening in the Arctic, with the so-called “Northwest Passage” opening up. And if the grim scenario of large port city flooding occurs in the future, shipping routes will change in ways impossible to predict today.
Scientifically, monitoring alterations to the soundscape in the world’s coastal ocean is important for understanding the myriad other changes it brings about, and for informing policy decisions.
Physical Oceanography Considerations
The Gulf of Maine and the southern New England shelf are among the most rapidly warming regions in the world ocean (Pershing et al., 2015). Warming in this region was highlighted in the first six months of 2012, when temperature anomalies were as large as 2.6°C south of New England and off Chesapeake Bay (Chen et al., 2014). The ocean temperatures over the continental shelf in 2012 were the highest recorded in the nearly 150 years of observations (Chen et al., 2015). The widespread temperature anomalies in 2012 were due to a Jet Stream ridge over the eastern half of North America that was persistent for a number of weeks during the winter. This local northward shift in the Jet Stream resulted in a reduction of the cooling rate for the continental shelf (Chen et al., 2014). In recent years, warming of the Arctic atmosphere has greatly affected the behavior of the Jet Stream (Francis and Vavrus, 2012), as illustrated in Figure 1a.
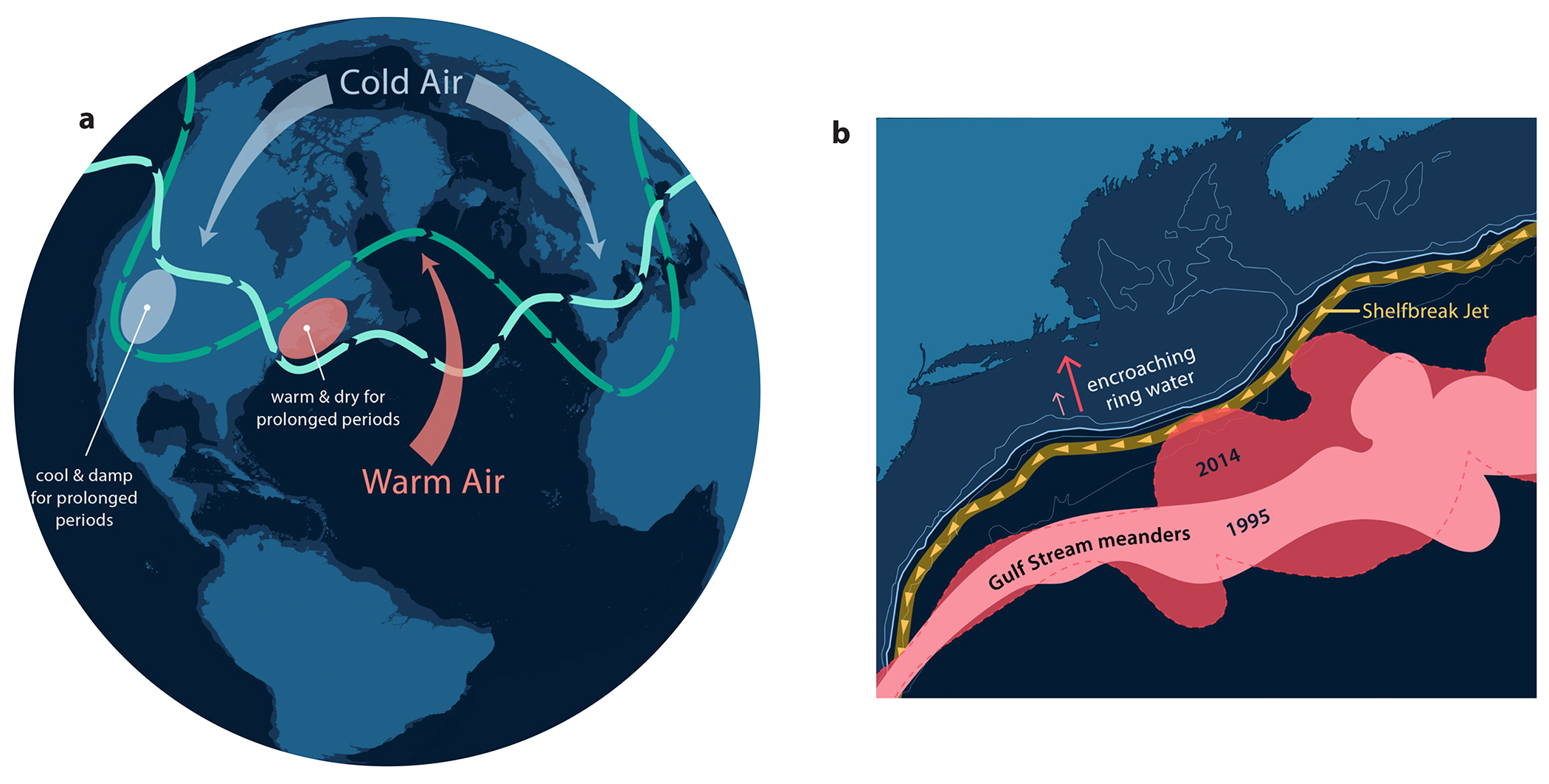
Figure 1. (a) A schematic of atmospheric factors relating to warming of the continental shelf in the northeastern United States. The Jet Stream is denoted by the green lines. Jet Stream meanders have in recent times increased in latitudinal extent (dark green versus light green lines). The larger amplitude meanders move more slowly, and are more susceptible to blocking events. This results in areas of prolonged warming (red oval) similar to the pattern in the winter of 2011–2012. (b) A schematic showing the increasing influence of the Gulf Stream on the continental shelf south of New England. The darker red pattern shows the Gulf Stream meander envelope in 2014, while the pale red pattern shows the meander envelope in 1995. In addition to the increasing proximity of the Gulf Stream to the shelf break, warm core ring intrusions penetrate further onto the continental shelf, denoted by the shoreward-directed arrows with the darker shade indicating recent years. The meander envelopes are taken from a figure in Andres (2016). > High res figure
|
The 2012 warming occurred over large spatial and temporal scales because it was driven by large-scale atmospheric processes that greatly altered air-sea fluxes during winter. Recently observed ocean advective processes involving onshore transport of warm continental slope water masses originating in the Gulf Stream have been implicated in generating large magnitude temperature and sound speed anomalies. Observations from both the National Science Foundation Ocean Observatories Initiative Pioneer Array and the Woods Hole Oceanographic Institution/Commercial Fisheries Research Foundation Shelf Research Fleet have identified several important shelf-break exchange events, most notably a warm core ring intrusion onto the continental shelf that extended more than 100 km onshore of the shelf break (Gawarkiewicz et al., 2018). The temperature anomaly from December 2016 through January 2017 was as large as 5°C, with a sound speed anomaly of 20 m s–1. Observations from gliders operated near the Pioneer Array indicated that temperatures over the continental slope in late January were 15°C, forcing the ring intrusion across the continental shelf. Recent analysis of Gulf Stream meander patterns (Andres, 2016) suggests that Gulf Stream meanders have both increased in amplitude and been initiated much further west over the past 20 years, bringing Gulf Stream water closer to the continental shelf south of New England (Figure 1b). This research shows the importance of recognizing that a number of different processes can generate large temperature and sound speed anomalies over the continental shelf and slope in the northeastern United States, and that they may occur much more frequently in the future.
The warming rates and extreme events over the continental shelves of the Arctic Ocean are less well understood. However, an important aspect of shelf/slope hydrography in the Arctic Ocean is the layering of cool, fresh shelf water masses over the continental slope (e.g., Melling et al., 1982). Specifically, a relatively cool, fresh surface layer extending to roughly the depth of the shelf break overlies a more salty, colder layer, the Arctic halocline, which in turn overlies the warm salty Atlantic water that rings the central Arctic Basin. This complex layering structure leads to important propagation effects relating to acoustic ducts. Sound energy becomes trapped in these ducting layers, and propagates with little attenuation. This is one way the changing nature of stratification in the water column in the Arctic, which is related to the loss of sea ice, is having profound effects on acoustic propagation across the continental shelf and slope in this region.
Mid-Latitude Acoustic Propagation Effects
Because water column temperature and salinity control sound propagation in shallow regions of the ocean, climate change effects will have a direct influence on underwater acoustics. Deepwater propagation is undergoing additional climate-related changes, in particular, from reduced volume absorption due to ocean acidification. However, seabed absorption usually dominates total absorption in shallow water, so the main focus here is on the likely effects of evolving sound speed from climate change, rather than on acidification effects.
Here, we address six reasonably likely mid-latitude ocean climate change effects for shallow water acoustics (Figure 2). These effects are: (1) water depth change, (2) impedance changes between the water column and the bottom, (3) shifts in positions and gradients within frontal regions, (4) closing of a near-bottom pathway of sound to deeper water, (5) wind and wave effects, and (6) the ocean soundscape (“noise field”). These effects are not as dramatic acoustically as the changing near-surface sound duct in the Arctic or the change in water column attenuation in deep water (i.e., abyssal depths) due to acidification. But they are expected changes, and deserve examination.
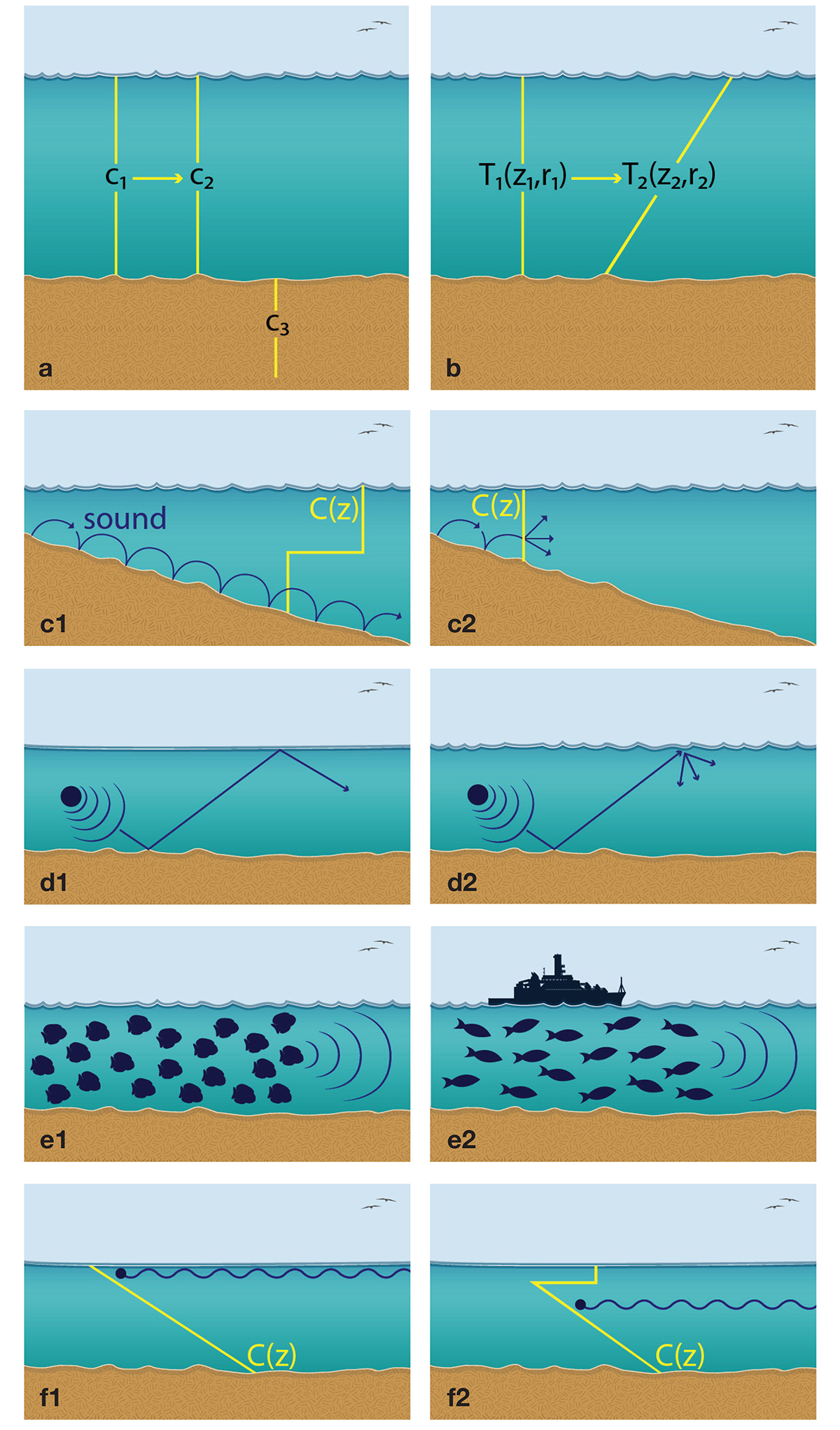
Figure 2. Possible effects of climate change on coastal acoustics. (a) Increase in water column sound speed that changes acoustic impedance contrast with the bottom. (b) Movement and change of shape of a coastal temperature front. (c1) Sound “flowing downhill” in a usual coastal downward refracting profile. (c2) Movement of a frontal feature blocking seaward propagation by scattering sound. (d1) Scattering from a relatively calm air-water interface. (d2) Increased (or decreased) surface sound scattering loss due to possible changes in wind patterns. (e1) Soundscape due to pre-change fauna and shipping. (e2) Soundscape due to changed fauna and shipping patterns. (f1) Pre-warming Arctic surface acoustic duct. (f2) Present Arctic subsurface duct, with better mid- to high-frequency acoustic propagation characteristics. > High res figure
|
Before explicitly looking at these effects, it is useful to briefly discuss how the ocean acoustic field relates to some important parameters, in particular, water depth, frequency, and sound speed. It is in this context that we can judge how much ocean climate change affects sound in the shallow seas.
The first parameter to look at is the acoustic wavelength, which in relation to the bathymetry sets the vertical scale of the system. The simplest acoustic dispersion relation, λν = c, will suffice for our purposes here, where λ is the wavelength, ν is the frequency, and c is the sound speed (in either the water or the bottom, depending on what is of interest). Sound speeds in the water column range from about 1,450 m s–1 to 1,550 m s–1, and in the top of the sediments (avoiding rock bottoms for now) from about 1,500 m s–1 to 2,000 m s–1. For simplicity, we can take 1,500 m s–1 as a typical continental shelf water column value, and 1,750 m s–1 as a typical sediment value. Sound frequencies typically used in ocean acoustics range from about 10 Hz to 10 MHz. If we look at what the wavelengths in water are for these two frequencies, we get from 150 m to 1.5 × 10–4 m (150 microns).
As mentioned, an important length scaling is the relation of the acoustic wavelength to the water depth. In particular, we are interested in regions where the water depth is order of 10 acoustic wavelengths. At around this point, where sound interaction with the seabed transitions from rare to frequent, there is a transition from low-frequency acoustics (which calls for use of a full wave equation treatment of the sound) to high-frequency acoustics (where a ray equation treatment is acceptable for many purposes.)
Given the vast range of frequencies that it is possible to examine, we need to restrict our discussion to some limited, but important, frequency ranges. Two good choices are: (1) 50–1,000 Hz, in which sound travels the farthest distances on the continental shelf (Jensen and Kuperman, 1983) and which larger cetaceans utilize, and (2) 1–100 kHz, which contains the vocalization and hearing frequencies of much of the marine life on the shelf. Both humans and aquatic animals make a lot of use of these two bands. We will call the lower band “low frequency” and the upper band “mid-frequency.” The higher frequencies (ν > 100 kHz) are also used by humans and other animals, but these lower two bands are a bit more common.
The first climate effect we consider, water depth change, is one that the general public is perhaps most aware of, but acoustically is not really much of a problem. By redefining where the shoreline is as sea level changes, the acoustics rescales with the new depth, and really all that needs to be done is to change the bathymetry charts. There is a grim acoustic “benefit” to this scenario for the lands that are flooded. One difficult problem in ocean acoustics is determining the bottom’s acoustic properties, and for the flooded lands, these should already be known from land surveys.
“Seabed mapping, anti-submarine warfare, conservation of endangered marine mammals, and the utility of acoustic beacons for industrial purposes and diver safety are all fundamentally affected by ocean warming and the associated changes in acoustic propagation and communication that result.”
The second climate effect we look at is due to the shifting thermohaline circulation, in particular, the observed shift inshore of the shelf break front or the extreme intrusion of eddies. A simple ocean plus bottom model, instructive for acoustic purposes, can be made of a frontal region, with the numbers we choose being representative. The water column and bottom are both considered to be isovelocity regions, with the water column sound speed shifting from 1,478 m s–1 inshore of the front to 1,510 m s–1 offshore of it. The bottom is taken as 1,750 m s–1. The front is considered vertical, another oversimplification, as fronts are often sloping. This type of ocean plus seabed model is called a “Pekeris model” (Pekeris, 1948) by ocean acousticians, in honor of Chaim Pekeris, who devised it for analyzing broadband shallow water data during World War II. It works reasonably well as a first-order calculation. There are two cases to consider from this model: first, what happens to sound propagating along either side of a front, and second, what happens to sound crossing a front?
Looking at sound on a given side of the front, let’s examine bottom grazing angles above and below the “critical grazing angle.” Above the critical grazing angle, sound propagates downward toward infinity in the bottom (in the context of this model), and so the energy can be considered lost. Below it, the energy is trapped in the water column waveguide, momentarily ignoring attenuation effects. The critical angle for the cold side of the front is found to be 29.6°, whereas on the warm side it is 27.3°. This gives a slightly larger angular sector on the cold side in which the sound is not totally lost. If we compare these, we see only about an 8% difference in the range of available propagation angles, not a huge amount. Of more interest is the angular regime below the critical grazing angle. For a lossless medium, this would be called the region of “total internal reflection,” where the sound is trapped and will propagate well along the waveguide. But the ocean bottom is far from lossless. (We ignore water column and surface and bottom roughness losses for now.) With a finite bottom attenuation coefficient, the acoustic energy penetrates the bottom in an exponentially decaying manner, with the exponential tail increasing in penetration with increasing grazing angle and decreasing impedance (the product of density and sound speed) contrast. Thus, if we consider a 10° grazing angle ray hitting the bottom on either side of the front, the one on the colder side will penetrate less into the bottom, and thus suffer less attenuation, than the same angle ray on the warm side of the front.
Of as much interest is the change in propagation loss due to the sound’s vertical angle interaction with the front. Frontal features scatter sound into a variety of vertical angles, with the exact distribution being determined by the acoustic frequency and the specific parameters of the front. Sound that scatters to higher grazing angles is quickly lost, so there is increasing loss after crossing a front. This is shown in Figure 2. We note that this Pekeris model example is strictly a pedagogical one, useful for discussion. Numerous acoustics papers have been written about sound propagation through real fronts, based on both acoustic and oceanographic data. Addressing the full range of variability of ocean fronts is beyond the scope of the current paper.
The fourth effect that is of interest is the closing of a sound pathway from a nearshore region with cooler water masses to the deep ocean sound channel. Consider (abandoning our isovelocity water column assumption for a moment) a front to have a certain vertical extent, from the surface to depth Hf, with cool water underneath, perhaps more typical of an intrusion than a shelf-break front. Also, let the shelf bathymetry have a seaward slope, that is, D = D(r), where D is depth and r is the offshore distance. If Hf < D(r), then the cold water can form a duct along the bottom, and sound can “flow downhill” until it reaches deeper water. Figure 3 shows an example of this effect. However, if the depth of the front is larger than D, then the near-bottom duct gets closed off.
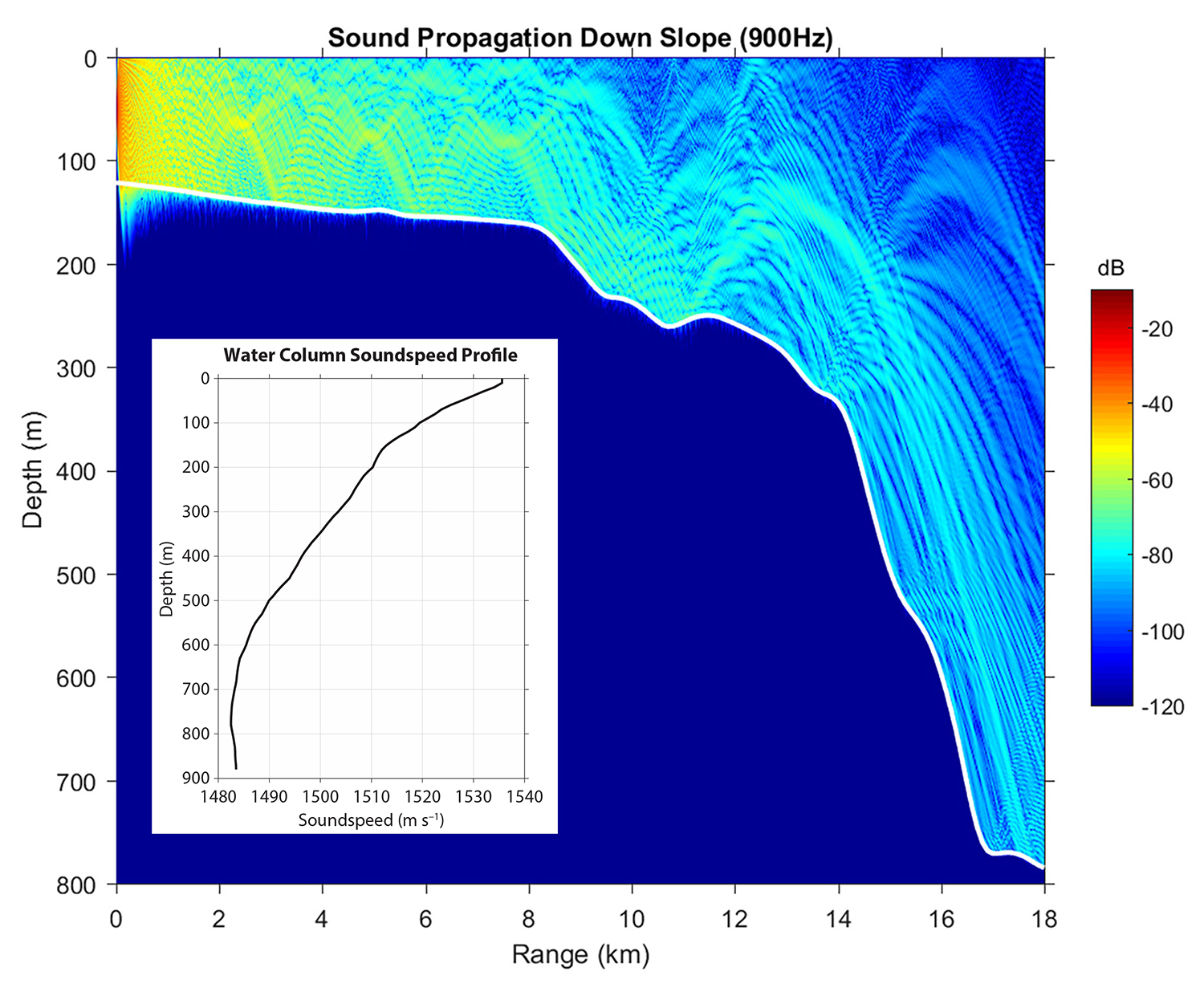
Figure 3. Sound appears to “flow downslope” due to cool, near-bottom waters overlain by warmer water above creating a downward refracting profile that makes the sound adhere to the bottom bathymetry. Example is from the East China Sea northeast of Taiwan. > High res figure
|
The fifth mid-latitude effect we consider is the effect of climatic changes on wind and wave patterns. There are two cases to consider here: mean patterns and storm events. The main concern for shallow-water acoustics is, of course, waves, as one of the “operational definitions” of shallow water is significant interaction with the surface and bottom boundaries. The wind effect of interest here is the creation of surface wave roughness (a first order acoustic propagation loss effect) and not so much the creation of noise (mainly due to the surface waves).
It is expected that climate change will bring about changes in the wind fields, with reduced wind speeds in some geographic areas (Karnauskas et al., 2018). This is favorable for shallow-water acoustic propagation, where surface roughness is a strong consideration. Currently, the prediction of acoustic attenuation effects for a given wind level, and thus surface roughness, is a well-developed area of acoustics. Acoustic scattering attenuation increases roughly exponentially as the wave field increases, up to a certain point determined by the “Rayleigh Parameter,” P = 2kσsinθ, where k is the acoustic wave-
number, σ is the root-mean-square surface roughness, and θ is the grazing angle (Brekhovskikh and Lysanov, 2003). Past this point, the scattering theory becomes more difficult, but there is a large acoustics literature available that addresses it.
Another mid-latitude climate consideration is storms, which are expected to increase in intensity as ocean warming continues. Storms create local ocean surface roughness and also distant swell, both of which are scatterers of sound in shallow water. They also create significant local noise. Prediction of acoustic effects versus storm intensity has also been studied (e.g., Shaw et al., 1978; Wilson and Makris, 2006), so the biggest question concerning acoustics is predicting increases in storm intensities as the ocean waters warm. Storm intensities over continental shelves are still difficult to understand and forecast (e.g., Glenn et al., 2015) and so future projections carry large uncertainty.
One interesting way of looking at the ocean’s acoustic field is via the “soundscape” concept (Miksis-Olds et al., 2018). The soundscape includes both natural and anthropogenic sounds, and can be used to give insight into ocean physics, anthropogenic activity, marine ecosystems, and how these vary in time and space. Another impact of ocean warming is the changing types of fish species in a given location. During a May 2012 Cape Hatteras experiment, there were significant changes in the types of fishes encountered, with schools of blue runners (Caranx crysos), a fish that favors warm water temperatures, predominating, rather than the typical species abundant in the spring such as bluefish and butterfish, which favor cooler water temperatures (Grothues et al., 2017). Large sound speed differences (up to 20 m s–1) were recorded during May 2012, as mentioned during the earlier physical oceanography discussion. To the extent that fish and other marine life produce sound, such a climate-induced change in species produces a change in the soundscape, which makes soundscapes an interesting way to remotely sense both climate change and shifts in ecosystems. Given that significant climatic changes are occurring, future studies should perhaps include a soundscape monitoring component. Doing so requires only relatively inexpensive hydrophones and recorders, and might be an interesting way to do both small- and large-scale coastal acoustic monitoring.
Arctic Shallow-Water Acoustic Effects of Climate Change
Before turning to Arctic acoustics, it is interesting to note that certain high-latitude areas may act acoustically somewhat like lower-latitude areas. Upper-layer temperature in areas such as the Mackenzie River plume may increase above the levels of 8°–10°C common a decade ago (Boeuf et al., 2013), and other areas that are commonly at 6°C in the summer may also show increases. With deep waters as cold as −2°C, a thermocline change of 12°C or more is not unlike what is seen in regions such as the Middle Atlantic Bight (Tang et al., 2007), where typical maximum late summer temperature differences are 15°C (9°–24°C, roughly speaking). Other areas such as the Bering Sea are also showing strong increases in summer surface temperature.
The Arctic Ocean has been undergoing dramatic changes, the most apparent and dramatic of which is its ice cover. Beginning in early 2000, the extent of multiyear ice covering the Arctic Ocean started decreasing significantly, and in 2012, sea ice extent reached the least ever recorded. With younger, thinner, and less ice on the sea surface, underwater sound experiences less transmission loss due to less reflection loss from the ice cover.
The other acoustically significant change in the Arctic Ocean is in the water column stratification at 100–200 m depth. Figure 4a shows two annual average profiles of water temperature, salinity, and sound speed in the Canada Basin from two different periods: (1) 1955 to 1994, and (2) 2005 to 2012. The data are from the World Ocean Atlas 2013 version 2 (Locarnini et al., 2013; Zweng et al., 2013) in a selected Beaufort Sea area (72°N~77°N, 145°W~155°W) in the Canada Basin. The comparison clearly shows that the so-called “Beaufort Lens” sound duct appears prominently in recent years due to temperature increases in both the Pacific Summer Water and Atlantic Water layers. Because this sound duct is between two water layers, mid- to high-frequency underwater sound waves can propagate for hundreds of kilometers inside the duct without interacting with the sea surface and seabed, as shown in Figure 5 (Freitag et al., 2015; Webster et al., 2015; Baggeroer et al., 2016; Carper, 2017; Duda, 2017). Figure 5 shows that this ducting effect carries from deep water, across the continental slope, and into the shallower coastal waters (Duda, 2017).
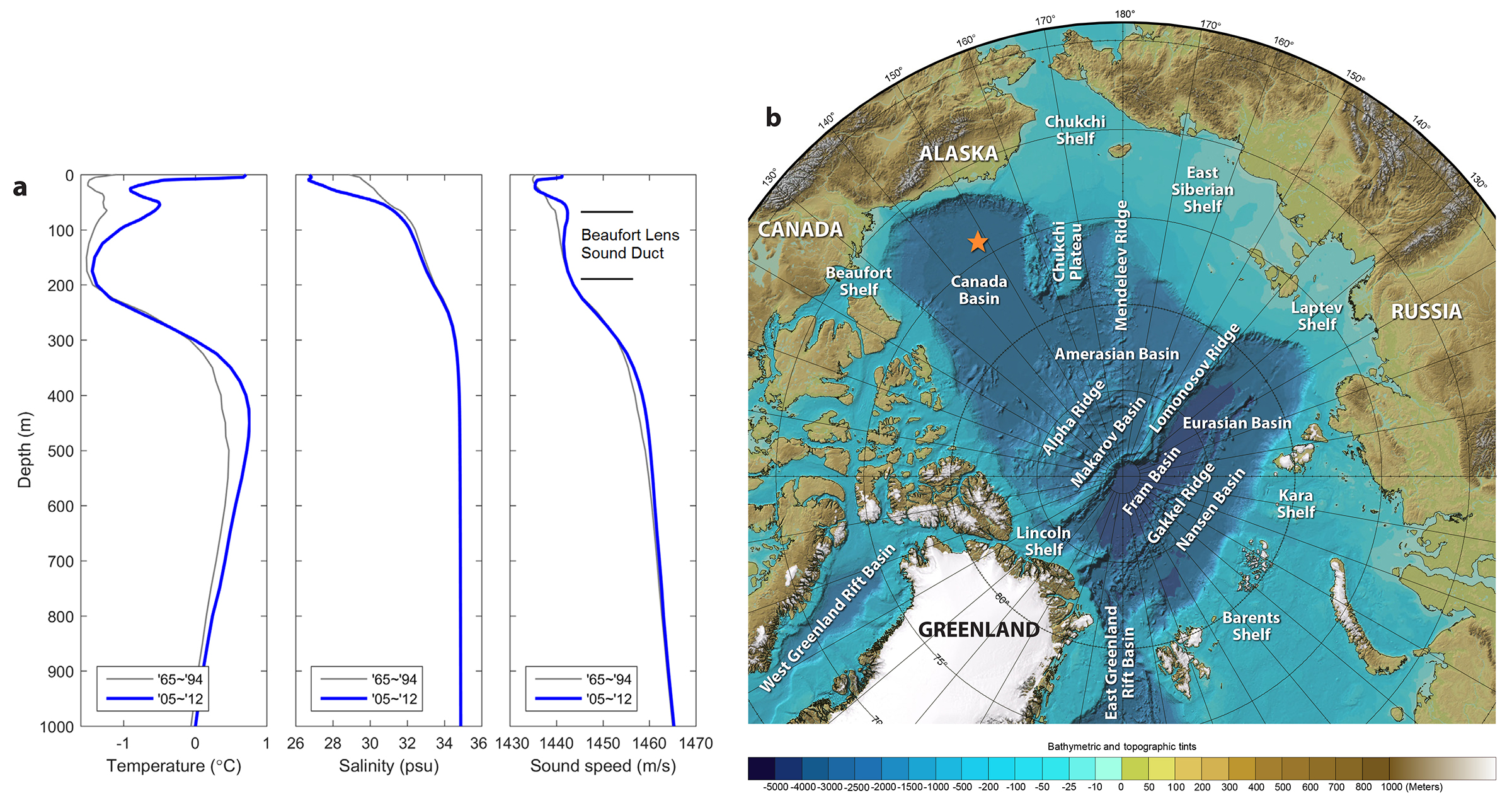
Figure 4. (a) Annual mean profiles of water temperature, salinity, and sound speed during two different periods in the Canada Basin, marked on the IBCAO bathymetric map (Jakobsson et al., 2012) shown in (b). The creation of a subsurface duct over time is readily apparent. > High res figure
|
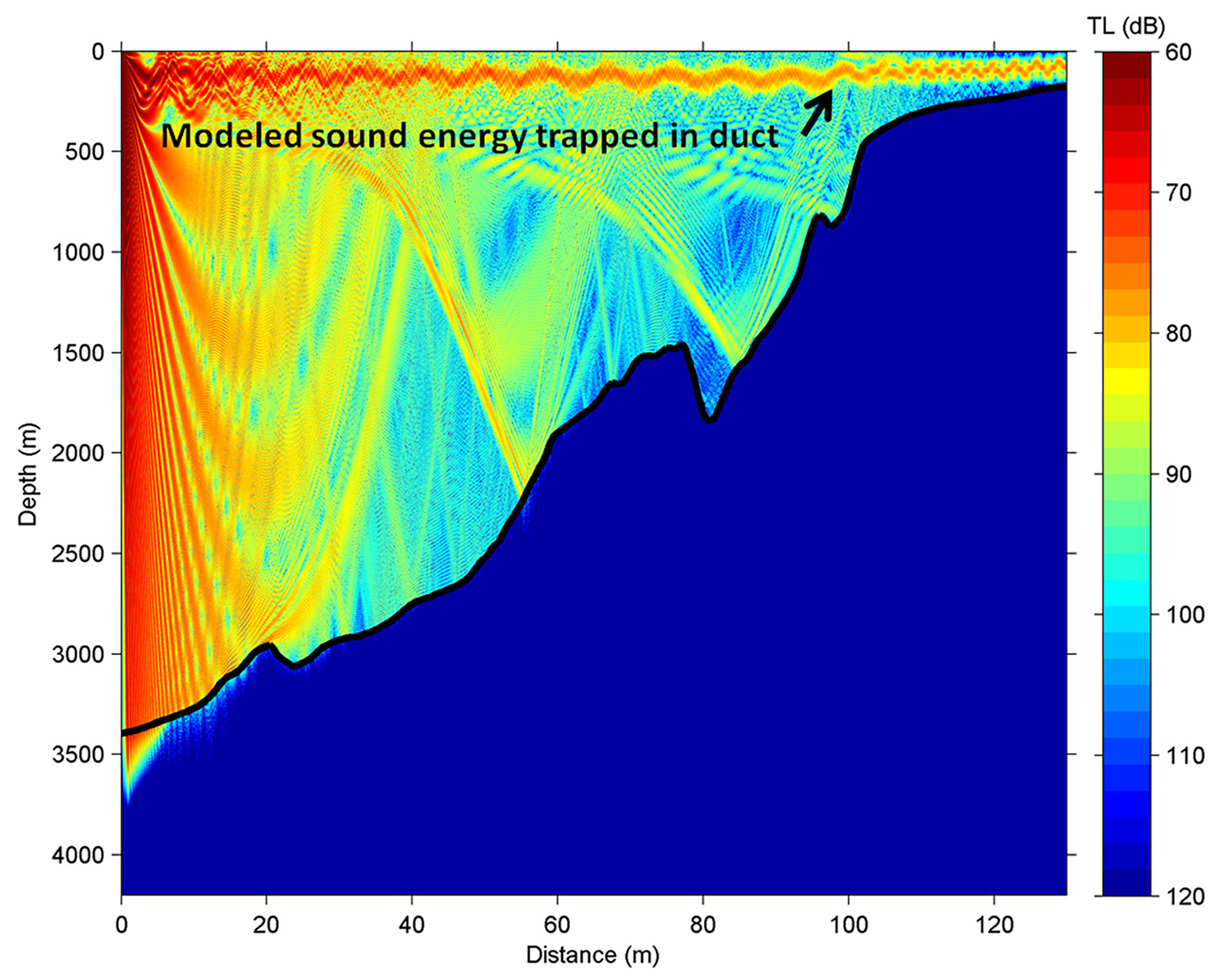
Figure 5. Numerical simulation of sound propagation through the “Beaufort Lens” sound duct from the deep Canada Basin onto the shallow Chukchi Sea shelf. The sound source is at 150 m depth, and the frequency is 250 Hz. The ducted sound does not interact with the surface (which can be either ice or water), resulting in much less propagation loss. > High res figure
|
Discussion and Conclusions
We have presented a rather general view of coastal acoustic effects of climate change that are likely to occur. Individual, detailed acoustic calculations will depend significantly on local conditions, given the high variability of shallow water regions. Predicting and measuring this oceanic variability for acoustics and other purposes will be a significant challenge for the entire oceanographic community.
We readily admit that the two example regions considered in this paper were drawn from our own research experiences, and that many other regional examples should be examined. However, the general mechanisms discussed, such as formation of new acoustic ducts, shifts in frontal location and gradients, and increasingly warmer waters should have broad applicability.
We have not done adequate justice to the biological changes that are occurring due to ocean warming, which will affect the ocean soundscape. While the northward migration (in the Northern Hemisphere) of warm-water species is well known (Pinsky et al., 2013), predicting the details of future spatial shifts is difficult. This would make an interesting part of a general underwater soundscape study. Also, predicting changes to the anthropogenic part of the soundscape (e.g., shipping route changes) is outside the acoustician’s usual toolkit, but is still crucial to acoustics and biology.
The overall discussion presented here has focused on the simple, fundamental physics of acoustic propagation and will need to be followed by a more extensive discussion among the scientific community about how to prioritize research on this broad topic. Seabed mapping, anti-submarine warfare, conservation of endangered marine mammals, and the utility of acoustic beacons for industrial purposes and diver safety are all fundamentally affected by ocean warming and the associated changes in acoustic propagation and communication that result.