Though geochemists have long sought to understand biogeochemical cycles, initial attempts to measure the extremely low concentrations of trace elements in seawater were frustrated by contamination problems. It was not until the 1970s that contamination-free methods were developed, launching a new era of research to characterize the biogeochemical cycles of trace elements in the ocean.
Nevertheless, by the beginning of the new millennium, after two decades of improved and, generally, contamination-free sample collection, work was proceeding so slowly that description of the marine biogeochemical cycles of most trace elements was beyond reach. For example, by 2003, dissolved iron (dFe) profiles from the surface ocean to >2,000 m had been reported for no more than two dozen locations worldwide (Anderson et al., 2014). Despite reliable data, for the most part, they were grossly inadequate to define biogeochemical cycling of Fe.
Efforts would have to be coordinated to characterize the global biogeochemical cycle of any trace element: no single nation, let alone an individual investigator, could hope to compile sufficient information. This recognition led to the creation of the GEOTRACES program (https://www.geotraces.org/), an international study of the marine biogeochemistry of trace elements and their isotope (TEIs).
The objectives of the program were straightforward: to determine ocean distributions of TEIs globally and to understand the processes that control them well enough to code the defining parameters into models. Achieving the necessary global coverage required contributions from many investigators in many nations, which, in turn, led to two further prerequisites: intercalibration, to ensure internal consistency of data generated by different labs (Aguilar-Islas et al., 2024, in this issue) and a data management system that combined the international suite of intercalibrated data into a single database that was available in multiple formats, including graphical illustration of the results in an electronic atlas (Schlitzer and Mieruch-Schnülle, 2024, in this issue). These prerequisites were put into place in advance of the global study.
International workshops held in 2007, focusing on the Pacific, Atlantic, and Indian Oceans, enabled investigators to identify target locations, either where strong sources or sinks of TEIs were thought to exist, or where internal cycling processes (biological uptake, regeneration, abiotic scavenging, transport by ocean circulation) have a strong influence over TEI distributions. Investigation of the Arctic and Southern Oceans began in 2007 under the International Polar Year (IPY). Although GEOTRACES was not ready at that time to undertake a full study of all TEIs of interest, the development of new technologies for the collection of contamination-free samples (de Baar et al., 2008) in preparation for GEOTRACES allowed some GEOTRACES investigators to participate in the IPY. More complete planning for Arctic Ocean work (Jensen and Colombo, 2024, in this issue) was organized during an international workshop in 2009. Workshop reports, containing recommendations for a global survey, are available at https://www.geotraces.org/planning-documents/.
A global survey of TEI distributions (Figure 1) was designed using the targeted locations noted above, enabling investigators to develop, and in some cases test, hypotheses concerning sources, sinks, and internal cycling of TEIs. The global survey is nearly complete. An interactive map with up-to-date information about GEOTRACES sections, as well as GEOTRACES process studies and the locations of compliant data (meets GEOTRACES standards of intercalibration), can be found at https://www.bodc.ac.uk/geotraces/cruises/section_maps/interactive_map/. By comparison with hydrographic data, the TEI data are still sparse, but they are adequate to convey a three-dimensional view of TEI distribution at the ocean basin scale, for example, dFe in Figure 2.
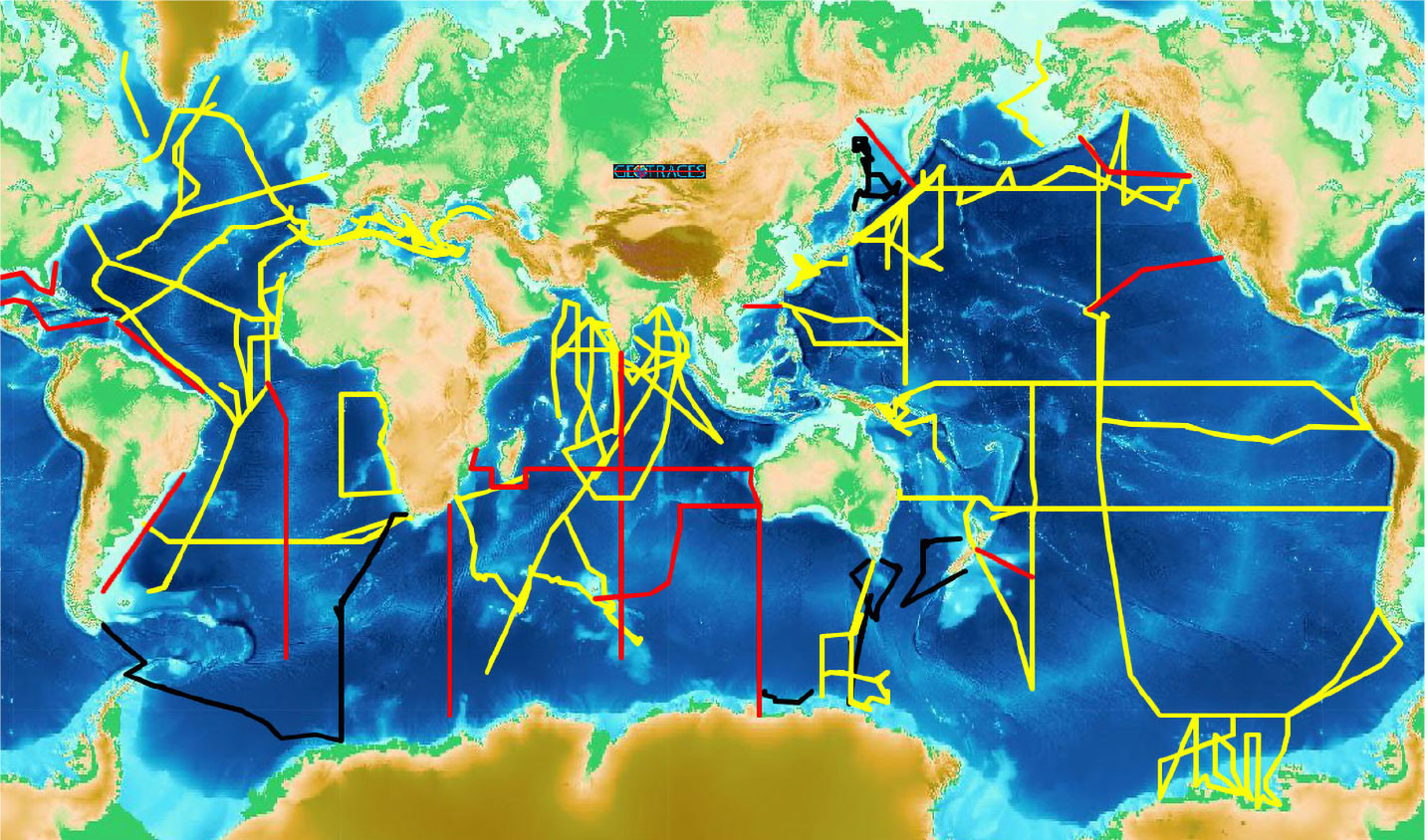
FIGURE 1. Status of the GEOTRACES global survey of trace elements and their isotopes, omitting the Arctic Ocean. Sections in black were completed as the GEOTRACES contribution to the International Polar Year. Sections in yellow were completed, as of February 1, 2024, as part of the primary GEOTRACES global survey. Sections in red are being considered but not yet completed. This figure is available from https://www.bodc.ac.uk/geotraces/cruises/section_maps/interactive_map/ where an interactive version is available with more information about each cruise. > High res figure
|
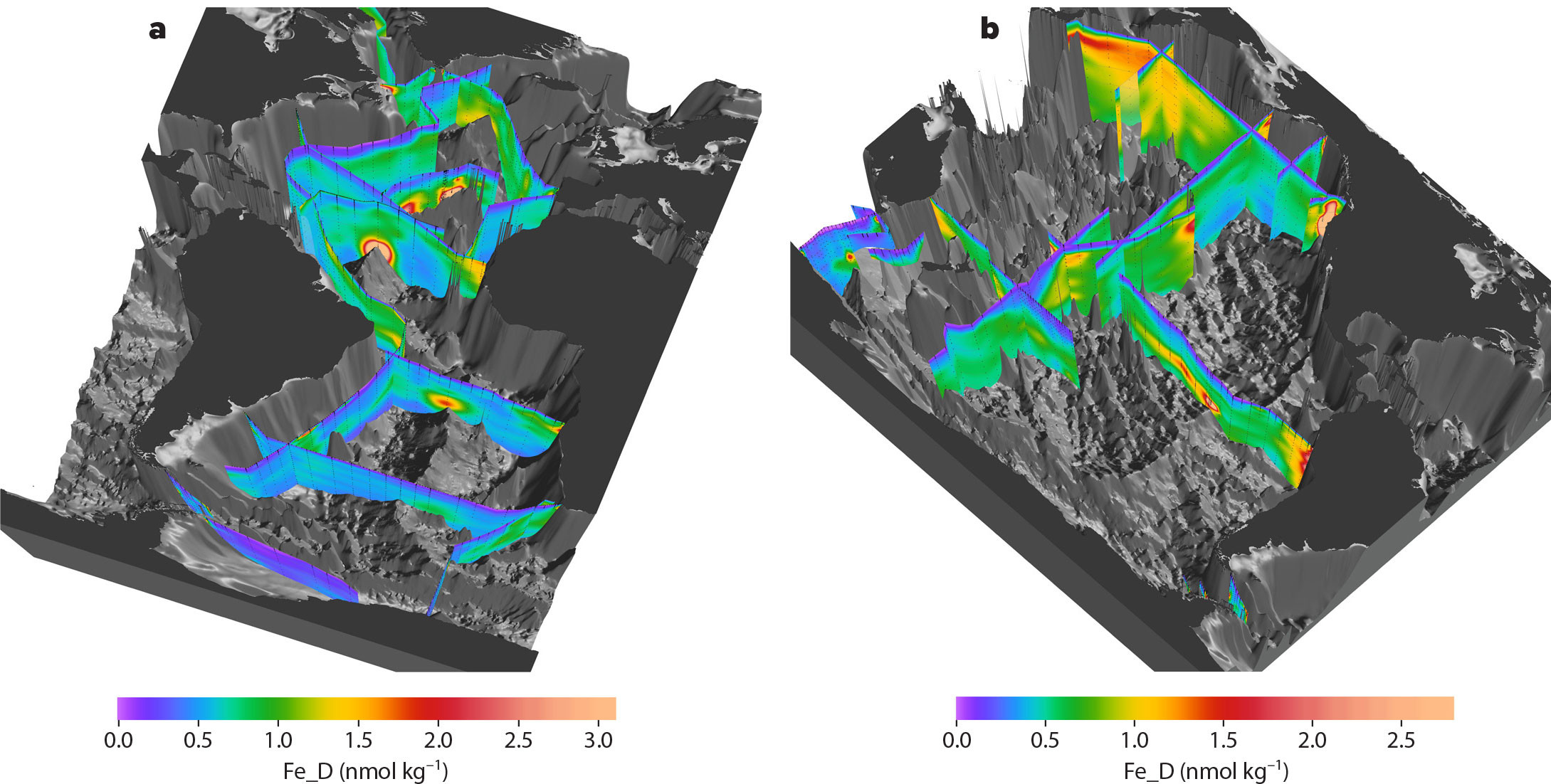
FIGURE 2. Dissolved Fe concentrations in the Atlantic (a) and Pacific (b) Oceans extracted from the eGEOTRACES electronic atlas (https://www.egeotraces.org/). Elevated concentrations of dissolved Fe are seen around the crests of mid ocean ridges, indicating hydrothermal sources, and near continental margins, indicating mobilization from sediments and from dust. Data are available in the GEOTRACES Intermediate Data Product IDP2021 (IDP, 2021). Images courtesy of Reiner Schlitzer, Alfred Wegener Institute. > High res figure
|
GEOTRACES has nearly completed the first part of its mission: to establish the distributions of key TEIs globally. However, much remains to be done to identify the processes that control TEI distributions, the rates of those processes, and their sensitivity to changing environmental conditions. As foreseen in the GEOTRACES science plan (GEOTRACES Planning Group, 2006), there is a need now for synthesis, which includes modeling and process studies.
Iron (see also Conway et al., 2024, in this issue) provides an example of new insights gained by GEOTRACES because, though essential for all organisms, it is so insoluble in oxygenated seawater that its low concentration, often <0.1 nmol kg–1 in surface waters, is thought to limit the growth of phytoplankton throughout much of the ocean (Browning and Moore, 2023). Furthermore, the distribution of iron is influenced by multiple complicating factors. For example, operationally defined dissolved iron (iron passing through a filter with a pore diameter of 0.2 µm or 0.4 µm) consists of both colloidal and soluble forms (the latter is thought to exist in true solution, although this is also operationally defined based on passing through a membrane with a nominal pore diameter; e.g., 0.02 µm). Soluble Fe exists almost entirely as organic complexes (Gledhill and Buck, 2012). Although the structure of the complexing ligands is unknown in most cases (Boiteau et al., 2016, 2019), there is growing evidence that humic compounds are important ligands (Dulaquais et al., 2023; Fourquez et al., 2023). Whitby et al. (2024, in this issue) provide a more in-depth review of trace metal speciation. Colloidal forms, the abundance of which varies spatially, may be either organic or inorganic and constitute about half of the operationally defined dissolved Fe in seawater (e.g., Nishioka et al., 2001; Wu et al., 2001; Bergquist et al., 2007; Boye et al., 2010; Fitzsimmons et al., 2017; Kunde et al., 2019; Lough et al., 2019; Homoky et al., 2021; Jensen et al., 2021; Tagliabue et al., 2022).
With multiple contributing sources (rivers, dust, hydrothermal fluids, and sediments), the total supply rate of iron is difficult to quantify (Tagliabue et al., 2017; Somes et al., 2021). Biological uptake of Fe, and abiotic scavenging, processes that remove Fe from the ocean, are both sensitive to the chemical speciation and physical form of Fe (e.g., Wang and Guo, 2000; Chen and Wang, 2001). These factors create large uncertainty in the rates of supply, removal, and internal cycling of Fe in the ocean, as illustrated by the two orders of magnitude spread of estimated residence times of dFe in the ocean among 13 models presented in the first iron model intercomparison project (Tagliabue et al., 2016). As these models were tuned to match observed dFe distributions, the range of residence times is a measure of the uncertainty in the rates of supply and removal.
Several studies have since attempted to improve global models of iron in the ocean (see Tagliabue and Weber, 2024, in this issue). For example, Somes et al. (2021) combined the University of Victoria Earth System Climate Model with the Model of Ocean Biogeochemistry and Isotopes (MOBI), incorporating estimates of iron supply by dust, by reductive sediment dissolution, and by hydrothermal fluids. They varied the inputs of Fe from dust and from sediments and used either constant or variable concentrations of Fe-binding ligands. For each scenario, scavenging (removal) rates were tuned to achieve an approximation of the observed dFe distribution. High rates of Fe supply and removal were required to match observed spatial gradients in dFe concentration. Consequently, average surface and global-ocean residence times of dFe were short, 0.83 and 7.5 years, respectively. This estimate for the global-ocean residence times of dFe is at the low end of the range obtained in previous models (Tagliabue et al., 2016), and much lower than the often-used estimate of deep-water scavenging residence time for dFe of 270 ± 140 years (Bergquist and Boyle, 2006).
Short residence times of dFe, which imply high rates of supply and removal, are supported by results from radionuclide-based methods employed in GEOTRACES. Investigators have used 7Be to estimate the supply of Fe from the atmosphere (Kadko et al., 2020), 234Th to estimate the export of Fe from the surface ocean (Black et al., 2020), and combined 230Th with 232Th to estimate the supply of dFe from atmospheric sources to surface waters (Hayes et al., 2015, 2017; Hayes, 2024, in this issue). While the surface-ocean dFe residence times estimated by these methods ranged widely (with season and proximity to productive upwelling systems), they were comparable to the average value generated by Somes et al. (2021). Likewise, full water column dFe replacement times estimated for the tropical North Atlantic Ocean by combining 230Th with 232Th were also at the low end of model estimates (four to eight years; Hayes et al., 2018), consistent with the global-ocean residence times of dFe reported by Somes et al.
Agreement between model estimates and radionuclide-based methods, noted above, provides incentive to combine radionuclides and trace element data in future synthesis efforts that aim to constrain rates of TEI supply and removal. Improvement can be expected as limitations of observational and modeling approaches are overcome. For example, the Somes et al. (2021) model does not contain non-reductive mobilization of dFe from sediments, or the release of colloidal Fe, both of which have been shown to be important components of the ocean Fe cycle (Conway and John, 2014; Homoky et al., 2021). Radionuclide-based methods, on the other hand, require assumptions about the solubility of Fe derived from aerosols (Kadko et al., 2020), about the lithogenic Fe/Al ratio (Black et al., 2020), and/or about the relative solubility of Fe and Th (Hayes et al., 2015, 2018). Future synthesis studies that address these assumptions will provide new insight into critical biogeochemical cycles.
New understanding of biogeochemical cycling has come from process studies as well as from the global survey. For example, time-series measurements at the Bermuda-Atlantic Time Series station (BATS) show a strong seasonal variability of dFe in surface water that is not accompanied by an equivalent variability of ligands (Tagliabue et al., 2023). This suggests that much of the dFe supplied during the summer dust maximum is removed by formation of authigenic colloids that then coagulate into larger, settling particles (Tagliabue et al., 2023), a process that was not considered in previous models. This shows how studies can be designed to address critical processes that cannot be extracted from global survey data.
While this article focuses on Fe, uncertainties about sources, speciation, and removal processes also limit our understanding of the marine biogeochemical cycles of other trace elements. For example, although it was established decades ago that most dissolved Cu in seawater exists as organic complexes (Coale and Bruland, 1990), it was recently discovered that much of the organically complexed Cu may be inert (unavailable for biological uptake or abiotic scavenging; Moriyasu et al., 2023). The possible existence of organically complexed or colloidal inert Cu was noted in previous GEOTRACES studies (Ruacho et al., 2020), and terrestrial sources of these chemical species have been suggested (Zitoun et al., 2021; Moriyasu et al., 2023), but the impact of these inert forms on the biogeochemical cycle of Cu has not yet been explored in models (Richon and Tagliabue, 2019; Roshan et al., 2020). Future studies that establish the nature and source(s) of these inert forms, as well as their impact on the marine biogeochemical cycle of Cu, will constitute a major advance of the field.
Synthesis in GEOTRACES has also led to some notable advances in our understanding of TEIs. For example, the strong correlation between dissolved Zn and dissolved Si has been known since the first reliable Zn data were produced (Bruland, 1980). However, the incorporation of Zn into diatom frustules cannot explain this correlation given their low Zn concentration (Ellwood and Hunter, 2000). An alternative hypothesis to explain the correlation centers on the mixing of deep waters in the Southern Ocean that are rich in both Zn and Si, combined with the strong biological uptake of both Si and Zn in Southern Ocean surface waters (Vance et al., 2017; de Souza and Morrison, 2024, in this issue). Some models largely reproduce the global correlation with elevated biological Zn/Si uptake ratios in the Southern Ocean (de Souza et al., 2018). However, other models (Roshan et al., 2018; Weber et al., 2018) also need to incorporate abiotic reversible scavenging of Zn to fit global observations of Zn distribution. Further modeling of GEOTRACES data confirms the importance of reversible scavenging while also explaining the vertical distribution of the isotopic composition of dZn (Sieber et al., 2023), illustrating the multiple steps involved in establishing the global distribution of TEIs, a process facilitated by the global and internally consistent data of GEOTRACES.
Other advances based on GEOTRACES findings are described in the papers that follow in this issue and elsewhere (e.g., Anderson, 2020). The wealth of data (Figures 1 and 2) has already resolved previously unanswered questions, but the combination of different observational methods together with different modeling approaches is leading to unprecedented opportunities. We welcome and look forward to continued synthesis of GEOTRACES results and to novel process studies that will contribute even further to our understanding of global biogeochemical cycles of TEIs.
Acknowledgments
RFA was supported by a grant from the US National Science Foundation (NSF OCE-2219888) and he notes no conflicts of interest. Reiner Schlitzer provided the images in Figure 2 that were extracted from rotating three-dimensional animations in the GEOTRACES electronic atlas. The international GEOTRACES program is possible in part thanks to the support from the US National Science Foundation (Grant OCE-2140395) to the Scientific Committee on Oceanic Research (SCOR).