Introduction
Environmental change occurs in the Arctic at a greatly accelerated rate, warming at twice the global average, with profound impacts on the marine sector. Most apparent is the rapid decline in summertime sea ice extent, which in recent years has been roughly half of what it was in the late 1970s (Stroeve and Notz, 2018; Meier and Stroeve, 2022, in this issue). This loss has been accompanied by a shift to a younger, thinner, more mobile pack (Lindsay and Schweiger, 2015; Kwok, 2018) and a lengthening of the open water season (Stroeve et al., 2014). These changes lead to shifts in the dynamics that govern atmosphere-ice-ocean interactions, with broad impacts that include changes in weather, circulation, ecosystems, and biogeochemistry.
The magnitude and speed of Arctic environmental changes pose significant societal challenges. Climate-related hazards include accelerated coastal erosion brought about by more energetic surface waves. Food security is threatened by ecosystem shifts and unreliable ice conditions that complicate subsistence hunting. Arctic communities are finding it difficult to adapt to rapid changes in the ecosystem services they rely upon. Moreover, these hazards are not confined to the Arctic, as Arctic change may contribute to severe weather patterns in the mid-latitudes (e.g., Francis and Vavrus, 2012). As sea ice decline offers new opportunities for shipping, fisheries, resource extraction, and tourism, increased human activity brings new risks that demand expansion of capabilities such as search and rescue and oil spill response.
Observations provide a foundation for understanding and predicting Arctic change, but the challenges associated with operating in this remote, difficult environment have made the region data sparse. Recent results based on satellite altimetry (see Morison et al., 2022, in this issue) illustrate the difficulties imposed by the sparsity of in situ measurements. Ice cover impedes access to much of the Arctic, biasing existing measurements toward summer when sea ice extent is at a minimum, and complicating the task of achieving broad, distributed geographic coverage. Instruments deployed on sea ice are constrained by the circulation patterns of the ice itself. Autonomous instruments operating beneath the ice cannot access GPS and satellite communications that modern oceanographic systems, such as the Argo float array (Riser et al., 2018), rely on for geolocation and data transmission. The dynamic nature of sea ice complicates collection of collocated measurements across ocean, ice, and atmosphere. Added to this are issues such as severe cold, high winds, icing, and polar bear attacks on equipment that limit development of untended ice-based systems capable of collecting sustained, year-round atmospheric measurements. These challenges often demand development of larger, more complex instruments, while sustained, distributed observing efforts call for systems that are lighter and less costly (as defined by the sum of hardware, operations, and logistics).
Arctic Ocean observing serves a broad range of stakeholder needs that in turn place strong requirements on spatial and temporal scope and scales, and on data delivery. These can be organized into three overlapping domains, each with distinct demands for observations that guide the choice of platforms and approaches (e.g., Lee et al., 2019): (1) long-range planning and policy, (2) strategy, and (3) tactics. Policy (Figure 1, green) involves use of data to understand environmental change and make long-range predictions that inform decisions regarding management of natural and built environments on decadal timescales. This need often dictates distributed measurements of large geographic scope, sustained over decades. Real-time data delivery is not important, but maintenance of long, consistent records is critical. Strategy (Figure 1, orange) involves use of environmental data to support activity at timescales of seasons to years. Observations focus regionally and might support modeling efforts that require regular, near-real-time delivery of distributed observations. Tactics (Figure 1, purple) is the domain of situational awareness and operational forecasting to support day-to-day activity, such as search and rescue, subsistence hunting, vessel routing, and toxic algal bloom detection. Measurements might be confined near areas of human activity, with a premium on rapid delivery of data and analysis products. Engagement of coastal communities in the conceptualization, planning, and implementation of observing activities can improve relevance and, given appropriate choices of technologies, might offer efficient, sustainable paths for operations.
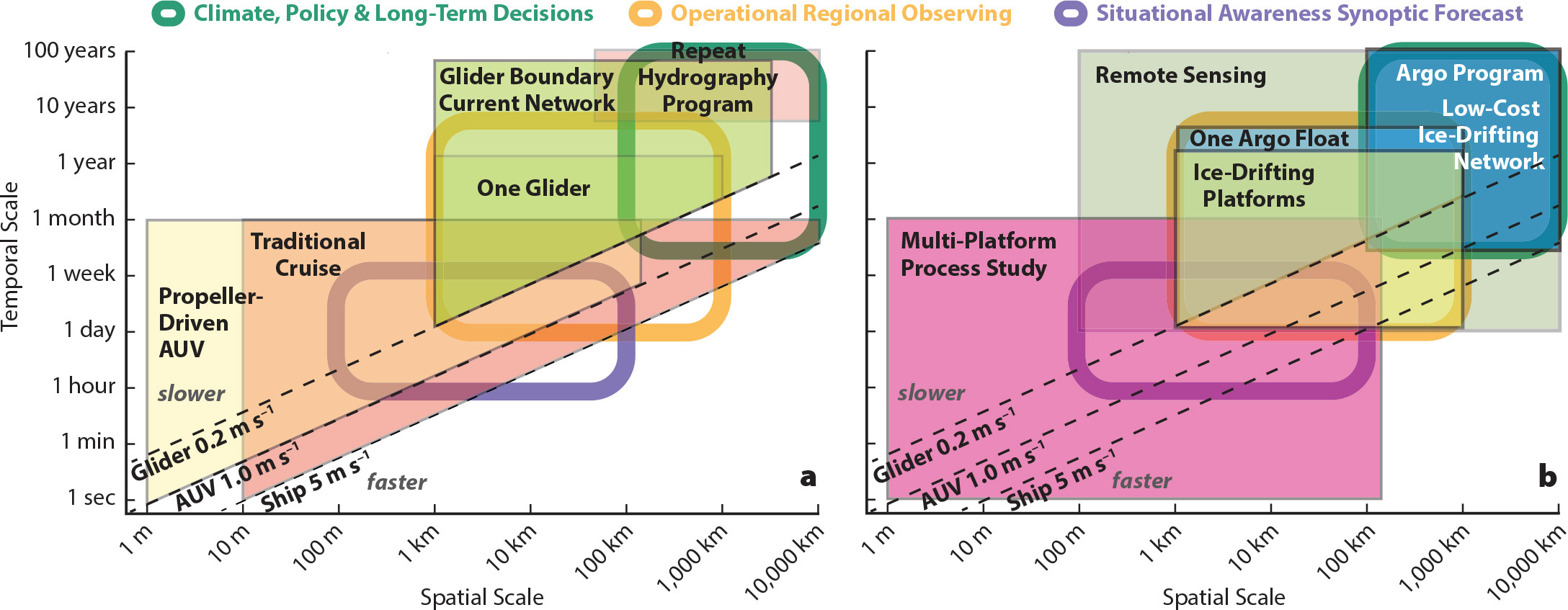
FIGURE 1. Spatial and temporal coverage provided by (a) mobile and (b) drifting platforms. Thick boxes mark the requirements of three notional observing systems. Lightly shaded areas denote scales sampled by selected platforms or networks of platforms, with the magenta box denoting the potential scope of a typical multi-platform process study. With the exception of remote sensing, all platforms shown provide vertical sampling though the water column. Maximum depth and ability to sample near the ice-ocean or atmosphere-ocean interface vary by platform. > High res figure
|
Ship-based surveys, camps established on the sea ice, and moored instruments have historically been responsible for most data collection in the Arctic Ocean. Ships and ice camps provide extensive in situ sampling capabilities and collect intricate measurements that are challenging or impossible to accomplish using the current generation of remote sensors. Moorings provide time series in locations such as shallow shelves and strong boundary currents that can be challenging for other platforms, and they can host large, power-hungry sensors that smaller platforms cannot accommodate. Moreover, ships remain critical for the deployment of moorings and small autonomous platforms. While ships and moorings will remain critical to Arctic observing, this paper focuses on exploring the capabilities provided by smaller autonomous platforms, including ice-tethered buoys, floats, autonomous underwater vehicles and gliders, and the sensors they support.
Emerging technologies, applications of existing technologies, and novel approaches for employing them offer paths for expanding Arctic Ocean observing to meet societal needs (Figure 2). Satellites provide long-term, pan-Arctic measurements of an expanding range of surface properties. New developments and ice-capable adaptations of autonomous platforms, including lightweight drifters, floats, and gliders as well as larger, more capable underwater, surface, and airborne vehicles, offer expanded geographic reach and persistence. Novel bio-optical, acoustic, image-based, and biogeochemical sensors, deployed on a range of ice-based, moored, and drifting platforms, promise to deliver key biological and chemical measurements at scales similar to those of physical properties. Advances in acoustic infrastructure provide new strategies for geolocation and data transmission for platforms operating beneath the ice. Used together, these systems can characterize spatial and temporal scales that have previously been difficult to achieve.
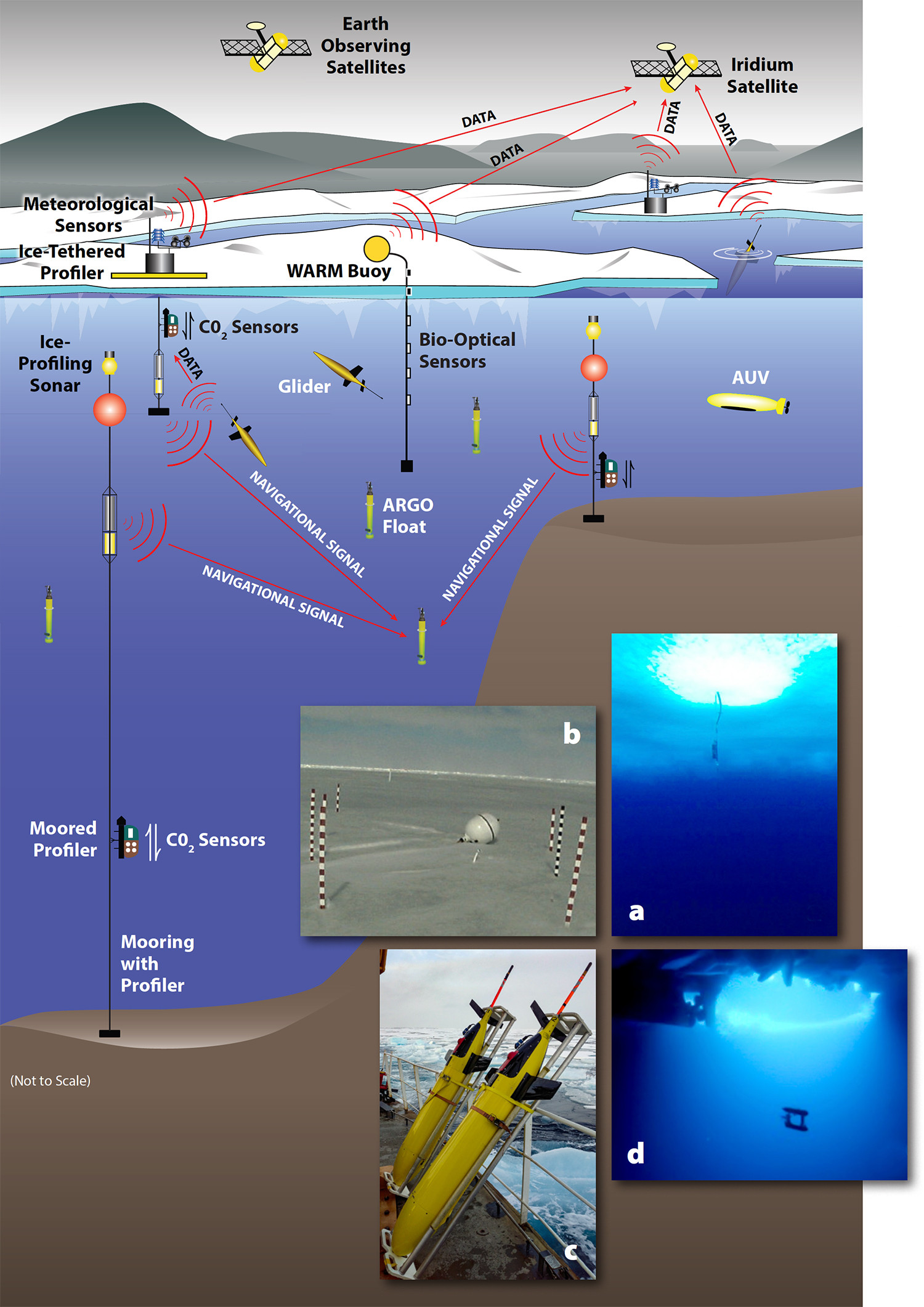
FIGURE 2. Technologies for autonomous Arctic Ocean observing. Red lines mark examples of underwater acoustic navigation and communication paths and satellite telemetry for instruments on the surface. (a) Sensors for measuring pCO2 and O2 deployed in the Beaufort Gyre on an Ice-Tethered Profiler. (b) A buoy designed for Warming and iRradiance Measurements (WARM). (c) Gliders ready for launch into the central Beaufort Sea. (d) The Jaguar autonomous underwater vehicle (AUV) deployed through an open lead for sea ice characterization. > High res figure
|
This paper focuses on autonomous platforms applied primarily to the deep, interior basins of the Arctic Ocean, and provides examples of emerging technologies, adaptations of mature technologies to the Arctic environment, and approaches for using heterogeneous systems of platforms to address challenges in Arctic Ocean observing. While many of these technologies can be readily used for collecting measurements across critical Arctic shelf systems, these shallow, ice-threatened environments pose unique, highly demanding challenges. Danielson et al. (2022, in this issue) provide an example of a collaborative Arctic shelf observing system that employs a diverse range of technologies and approaches, including some of the autonomous platforms discussed here. Recent insights enabled by satellite remote sensing reveal shortfalls in existing in situ observing capabilities (see Morison et al., 2022, in this issue). Here, this motivates a review of selected technologies for in situ observing that highlights challenges and promising new developments, followed by a discussion of emerging directions in sensor development. The paper concludes with recommendations stemming from ongoing development efforts.
Autonomous Platforms for In Situ Arctic Ocean Observing
The spectrum of Arctic Ocean observing systems (Figure 2) provides a framework for considering a range of mature and emerging technologies for autonomous in situ observing. The following discussion presents an overview of the state of the art of key platform classes, drawing on examples of emerging technologies to illustrate instrument capabilities and challenges.
Distributed Observations from Ice-Tethered Platforms
Ice-tethered systems have long been the backbone of autonomous seasonal and interannual observations of physical structure of the upper Arctic Ocean. Instruments such as Ice-Tethered Profilers (ITP; Figure 2a; Krishfield et al., 2008), the Autonomous Ocean Flux Buoy (AOFB; Figure 3a, Stanton et al., 2012), and Ice Mass Balance Buoys (IMB; Richter-Menge et al., 2006; Perovich, 2022, in this issue) routinely sample for periods of months to years while suspended from drifting sea ice, geolocating and transmitting data in real time using satellite services. Increasingly, changes in seasonal ice cover have made it more difficult to find large, stable ice floes that maximize instrument lifetimes, motivating efforts to harden systems to improve survivability during melt out and freeze-up, and to make open-water deployments possible. Drifting instruments, both on the ice and within the water column, can be highly effective for process investigations, but challenging to use for sustained, regionally focused observing networks in energetic environments, where ice drift and currents can rapidly carry instruments away from the region of interest. The combined cost of hardware and deployment logistics can make large-scale deployments impractical, limiting the utility of ice-tethered systems for broad, distributed observing. These challenges aside, ice-tethered platforms complement capabilities provided by other approaches and remain a critical tool for in situ observing at all scales. The Dynamic Ocean Topography (DOT) buoy, Warming and iRradiance Measurements (WARM) buoys, and Atmospheric Surface Flux Stations (ASFSs), each described below, provide examples of emerging developments that extend the utility of these established technologies into other applications.
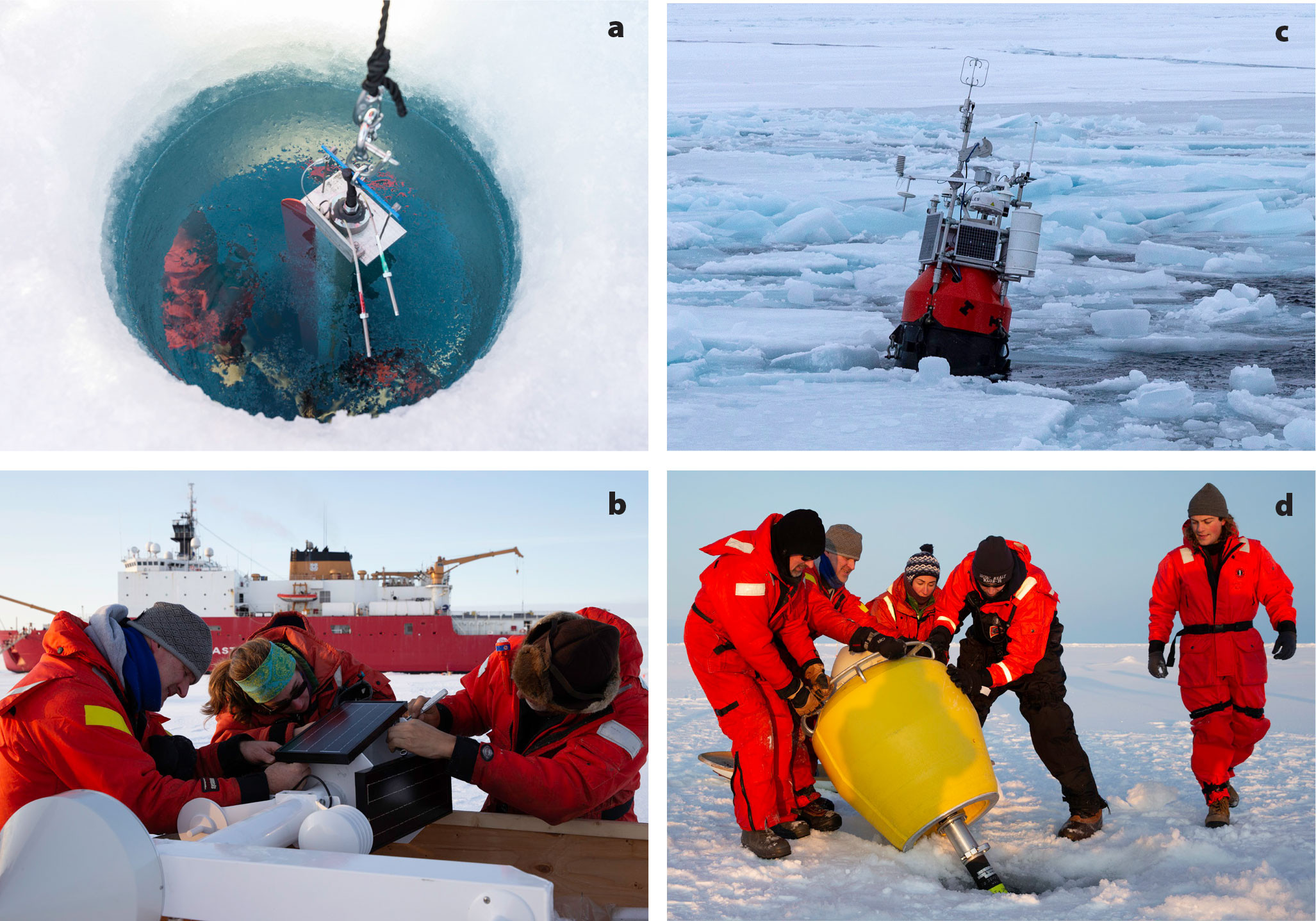
FIGURE 3. (a) Autonomous Ocean Flux Buoy (AOFB) being deployed at part of the 2018 Office of Naval Research (ONR) Stratified Ocean Dynamics of the Arctic Ocean (SODA) program. (b) Deployment of a Dynamic Ocean Topography (DOT) buoy from USCGS Healy in 2018. (c) Ice Gateway Buoy equipped with meteorological sensors drifting in the central Beaufort Sea during the 2021 ONR Arctic Mobile Observing System (AMOS) program. (d) Deployment of an acoustic navigation source. > High res figure
|
Dynamic Ocean Topography Buoy
The DOT buoy (Figure 3b) was developed to make precise measurements of sea surface height (SSH) and precipitable water vapor (PWV), aimed at providing the long-duration, in situ measurements needed to validate remotely sensed estimates of SSH, such as the NASA ICESat-2 and Surface Water and Ocean Topography (SWOT) altimeter missions (see Morison, et al., 2022, in this issue). Dynamic ocean topography, derived from SSH, constitutes the surface pressure gradient that drives geostrophic surface velocity, Vgeo. DOT observations combined with density profiles measured by Argo floats or ITPs to infer velocity shear allow estimation of absolute water velocity versus depth. In ice-covered seas, except during high winds, the sea ice drift, Vice, largely follows Vgeo (Kwok et al., 2013). The difference between Vice and Vgeo plays a critical role in stabilizing the doming of the Beaufort Sea Gyre (Dewey et al., 2018). Furthermore, cross-shelf gradients in DOT drive secondary circulations, upwelling, and downwelling, and are responsible for shelf-basin exchanges that are critical to maintaining the Arctic Ocean’s cold halocline (Morison et al., 2012).
The DOT buoy provides full GPS data to allow Precise Point Positioning determination of SSH with centimeter-scale accuracy. The resulting in situ SSH data are suitable for validation of ICESat-2 observations over long periods of time. The buoy resolves major tidal constituents as well as other high-frequency SSH variability unresolvable from remote sensing. A dual frequency GPS also allows the calculation of integrated PWV based on the wet zenith delay. A pressure sensor measures the freeboard of the GPS antenna phase center, a barometer measures atmospheric pressure to allow correction for the inverse barometer effect, and air temperature is measured for use in the determination of PWV. Standard meteorological measurements further augment the existing International Arctic Buoy Program (IABP) array in the Arctic Ocean. Data are telemetered through an Iridium link.
One DOT buoy was installed in Elson Lagoon, Alaska, in spring 2019 for comparison with an ICESat-2 target-of-opportunity. It was redeployed in the Beaufort Gyre in September 2019. A second buoy was deployed in the Eurasian Basin in October 2019 as part of the Multidisciplinary drifting Observatory for the Study of Arctic Climate program (MOSAiC; Shupe and Rex, 2022, in this issue). Both buoys survived for seven months. ICESat-2 photon heights from the target-of-opportunity pass on April 18, 2019, were within 0.003 m of the buoy-measured surface heights at the time of the pass and 0.02 m below the one-hour average of buoy-measured height, indicating the inherent accuracy of the buoy and ICESat-2 is at the 2 cm level. A third DOT buoy was deployed in spring 2022 in the Beaufort Sea by the Navy Ice Exercise (ICEX 2022) and is still reporting as of this writing.
Biogeochemical Measurements from Buoys
Integration of biological sensors onto ice-tethered platforms has enabled autonomous investigations of biogeochemical processes. Drifting with multiyear ice, ice-tethered systems equipped with sensors for light, chlorophyll and dissolved organic matter fluorescence, and backscatter at 700 nm have provided detailed vertical profiles several times a day. These data reveal differences in the vertical extent of algae biomass driven by euphotic depth across Beaufort and Central basins. Large aggregates of particles, assumed to be biological in nature, were observed sinking out of the euphotic zone, providing insight into sequestration of carbon in the central Arctic (Laney et al., 2014, 2017).
The WARM buoy (Figure 2b) was designed as a cost-effective ice-tethered platform to collect bio-optical observations throughout the melting phase of seasonal ice on the Arctic shelves. WARM buoy instruments include light, chlorophyll and dissolved organic matter fluorescence, backscatter at 532 nm, temperature, and salinity (Hill et al., 2018, 2020). In comparison to previous bio-optical systems, the sensors are static, swapping vertical resolution for the ability to collect measurements within the ice, at the ice-water interface, and within several meters of the bottom of the ice. Frequency of observations can be high and is limited only by battery life and satellite uplink. WARM buoys have observed under-ice phytoplankton blooms as well as intense and long-lived ice algal blooms in the Chukchi Sea (Hill et al., 2018).
Another novel technology for collecting observations close to the underside of the ice is the pop-up buoy, which is initially tethered to a bottom mooring and then released in the spring to nestle at the base of the ice and collect temperature, light, and chlorophyll fluorescence. These buoys record their data and then transmit via satellite uplink once the ice above them melts. Pop-up buoys deployed with Chukchi shelf moorings have observed dense, under-ice blooms in May on the ice-covered shelf (Stabeno et al., 2020). All of these systems have provided critical observations of conditions within and underneath the ice cover, observations that are critical to our ability to predict climate-related changes in Arctic primary productivity.
Mobile Platforms Operating Under the Ice
Small, long-endurance, mobile autonomous platforms, including profiling floats, surface drifters, autonomous surface vehicles, and buoyancy-driven gliders have expanded open-ocean observing to cover spatial and temporal scales that were previously difficult or impossible to resolve, but they have seen limited use in the Arctic. Relatively low hardware cost and light weight, flexible logistics make these platforms highly scalable and thus well suited for a wide range of observing tasks. Shallow water and challenging bathymetry complicate operation of gliders and profiling floats in the ice-covered nearshore, where long-range acoustic navigation is difficult to accomplish. The challenge of achieving year-round, real-time data return from instruments operating beneath the sea ice limits their utility in observing systems focused on serving tactical needs (Figure 1). Recent advances in profiling floats, long-endurance gliders, and the acoustic infrastructure that facilitates their operation in ice-covered environments will enable broader application of these platforms.
Profiling Floats
Profiling floats, which have revolutionized global ocean observing through the Argo program (Roemmich et al., 2009), offer a promising path for achieving sustained, distributed observations across the Arctic Ocean. Argo relies on low per-unit cost (~$25k for CTD-only units) and flexible logistics to maintain an operational network of nearly 4,000 floats that provide profiles at 300 km/10-day resolution across the ice-free oceans. Argo floats are a mature technology, mass-produced by several manufacturers, and have well-quantified reliability statistics. Because these floats can be deployed from vessels of opportunity or dropped from aircraft by personnel with little specialized training, the Arctic community’s long-standing culture of collaboration could provide many opportunistic deployments from research and commercial vessels, and aircraft-based deployments through operations such as the US Coast Guard’s routine reconnaissance flights.
Instruments operating beneath the ice cannot access satellites to geolocate and communicate, and must instead rely on alternative solutions, such as acoustics, or operate without. Previous efforts have successfully demonstrated under-ice acoustic navigation for extended (weeks to months) glider missions (e.g., Webster et al., 2014), which could be readily transferred to floats. Existing technologies for high bandwidth acoustic communications have ranges of only kilometers, but dramatic reductions in summertime sea ice extent offer large areas of seasonal open water, raising the possibility that floats could transfer data via satellite during the summer months. Within the framework of a coupled ocean-sea ice state estimate, Nguyen et al. (2020) find that even when floats are allowed geolocation and data transfer only when operating in low ice concentrations or in open water, the resulting data could still be useful for constraining numerical models and reducing hydrographic uncertainties.
Pilot efforts have achieved successful profiling float deployments in polar regions. As part of NOAA’s Arctic Heat experiment, Air-Launched Autonomous Micro Observer (ALAMO) profiling floats (Jayne and Bogue, 2017) deployed in late summer 2016 successfully sampled through the winter, and resurfaced in open water in spring 2017, though data transfer was limited due to the choice of a short surface interval (Wood et al., 2018). Development of acoustic geolocation and ice avoidance for floats operating in the Antarctic (Klatt et al., 2007) demonstrates successful implementation of these technologies on profiling floats and provides guidance for efforts to move these capabilities into the Arctic.
Long-Endurance Gliders
Buoyancy-driven gliders (Figure 2c; Lee and Rudnick, 2018) complement the sampling capabilities of profiling floats, resolving scales of kilometers and hours, while offering persistent sampling over seasons to years (Figure 1). Per-profile costs are similar to those of floats, but glider profiles are finely spaced along transit lines rather than distributed over broad regions. This makes gliders well suited for sustained sampling of regions with strong lateral contrasts, such as boundary currents, fronts, and eddies, and for capturing episodic processes that unfold at small scales. Long-endurance gliders are a mature technology with a record of scientific achievements (Rudnick, 2016), but they have the same geolocation and communication challenges as profiling floats when operating in ice-covered regions.
Multi-month missions under ice require extending the existing capabilities of gliders. Unlike floats, gliders actively navigate between commanded waypoints and thus require real-time geolocation. Implementation of long-range, low-frequency (1 kHz) acoustic navigation, starting with the system originally used for Ranging and Fixing of Sound (RAFOS) floats (Rossby et al., 1986) and onboard multilateration algorithms fulfilled this need for vehicles working in polar regions. Existing underwater gliders communicate to shore multiple times each day, and in some sense could be considered high-latency remote control vehicles. Seagliders operating in polar regions might go for many months without human intervention and thus require approaches and programming that provide extended autonomy, including navigation, mission decision-making, onboard troubleshooting, and ice avoidance. Though an inadequate substitute for satellite communications, high frequency (~10 kHz) acoustic communications provide limited through-water data transfer at ranges of a few kilometers.
Long-endurance Seagliders, geolocating from low frequency (~1 kHz) acoustic sources, have been used for multi-month missions under sea ice in the Arctic and Antarctic. Initial efforts focused on achieving year-round occupation of a section across the seasonally ice-covered Davis Strait (Curry et al., 2014; Webster et al., 2014). The limited 300 km-wide domain, Greenland-based local logistics, and year-round open water on the eastern edge of the domain made this an excellent test bed, with Seagliders first achieving a continuous year of sampling in 2010–2011. As part of the 2014 Office of Naval Research (ONR) Marginal Ice Zone (MIZ) Experiment (Lee and Thomson, 2017), Seagliders occupied sections from deep in the pack to open water, spanning the difficult-to-sample marginal ice zone in the central Beaufort Sea. MIZ gliders navigated from acoustic sources suspended from sea ice, sampling in the drifting reference frame of the ice to maintain collocation with ice-based instruments over a melt season. Most recently, Seagliders have executed missions lasting more than 14 months, operating under ice without communications for periods of up to 10 months near and beneath the Dotson ice shelf in the Antarctic and in the central Beaufort Sea.
Autonomous Underwater Vehicles
Autonomous underwater vehicles (AUVs; Figure 2d) have, over the last three decades, begun to be deployed routinely in polar regions. They have been used for a variety of commercial, military, and scientific under-ice applications, including laying fiber-optic cables (Ferguson, 1998), bathymetric surveys (Kaminski et al., 2010), surveys of the undersides of sea ice (Williams et al., 2015) and ice shelves (McPhail et al., 2009), and for geological and biological surveys at mid-ocean ridges (Sohn et al., 2008).These vehicles are characterized by the lack of a tether and by the fact that they carry relatively sophisticated payloads while maintaining an ability to work in harsh and complex environments with little or no human intervention. AUVs span a broad range of sizes and capabilities, dictated by mission requirements (e.g., Butler et al., 1993; Allen et al., 1997; Furlong et al., 2012).
Extended missions under sea ice require advanced capabilities, such as increased efficiency and the ability to hibernate under the ice to lengthen endurance. Accurate, precise navigation, central to many missions, will depend upon long-range external navigation beacons (e.g., Figure 3d), the use of inertial navigation solutions, or developing techniques to identify leads and then surface and submerge within them. Approaches for mid-mission data transfer mitigate the risk of vehicle loss during extended under-ice deployments. Short range and limited bandwidth render underwater acoustic data transfer impractical, making novel solutions like in-ice docking stations for data and power transfer critical. The partitioning of docking infrastructure between the AUV and the dock has been optimized (Singh et al., 1997), and further advances include the possibility to accommodate multiple vehicles, of different sizes and shapes, at the same dock. The emergence of large buoys designed for use in Arctic sea ice and capable of significant power storage should pave the way for docking technology to progress.
While efforts are underway to conduct autonomous manipulation tasks with AUVs (Fernández et al., 2013), advances in hybrid remotely operated vehicle (ROV)/AUVs (Bowen et al., 2009) have allowed for the physical collection of samples from the ice-water interface and from the seafloor in the ice-covered regions. These vehicles can serve as regular AUVs, but then, using single-use fiber-optic tethers, can be converted into tethered vehicles that allow operators to perform manipulation tasks.
AUV technological innovation, similar to what has taken place in the commercial small-drone community, is needed if AUVs are to become available to a wider user group. These innovations would ideally provide smaller, robust, easy to operate/deploy/recover and logistically light-weight vehicles. This would open up monitoring and repeated sampling opportunities for local communities and non-scientific uses alike, allowing broader use of AUVs within a sustained Arctic observing network.
Acoustic Navigation
Efforts to enhance the performance of acoustic navigation for platforms operating in polar waters focus on improving accuracy, adding flexibility, and increasing range. Broadband signals have been demonstrated to reduce position errors by an order of magnitude in open water applications (900 m to 80 m, root mean square; Van Uffelen et al., 2016). Broadband signaling has been successfully implemented for acoustic navigation of Seagliders operating in the Arctic, providing reductions in position error and the ability to encode source position and a small command set onto the navigation signal (Freitag et al., 2015). The ability to include source position with each broadcast allows the use of mobile navigation sources and provides increased flexibility in the design of navigation networks. Surface ducting and the resulting reflections off the rough ice-ocean interface limits the range of low-frequency acoustic navigation signals. This can be overcome by using very low frequency signals (~10 Hz) whose wavelengths are much longer than the roughness scales of sea ice (e.g., Gavrilov and Mikhalevsky, 2006). At the time of writing, programs supported by the US Office of Naval Research are testing a very low frequency navigation system aimed at providing basin-scale geolocation from a modest number of acoustic sources. Arctic acoustic networks could also be enhanced to provide other critical measurements, including integrated ocean heat content through acoustic thermometry and ambient ocean sound (e.g., Mikhalevsky et al., 2015).
Sensor Technology and Arctic Deployment Strategies
Opportunities/Challenges with Biogeochemical Sensor Technology
Ideally, autonomous biogeochemical sensors could be deployed on the various platforms described in the earlier section on Autonomous Platforms to quantify air-sea CO2 fluxes, the biological pump, and ecological structure and function. Biogeochemical sensors, however, are only just beginning to be deployed on a scale similar to physical sensors (Bushinsky et al., 2019). Sensors for dissolved O2, pCO2, pH, nitrate, and bio-optics (backscatter, chlorophyll fluorescence) are mature technologies (Wang et al., 2019), while in situ prototypes have been demonstrated for dissolved inorganic carbon (Fassbender et al., 2015), total alkalinity (Spaulding et al., 2014), and phosphate (Mowlem et al., 2021). Many of these systems are more analyzers than sensors, with pumps, reagents, and associated plumbing. Complexity, cost, and size scale accordingly, and these systems are challenged to achieve laboratory levels of precision and accuracy. While also complex, in situ instruments for quantification of planktonic populations (e.g., flow cytometry) have provided a wealth of information on plankton dynamics (Hunter-Cevera et al., 2016). However, their large data bandwidths and power requirements generally limit them to cabled, moored observatories (Boss et al., 2022). More affordable modular plankton imaging technology (PlanktonScope) with low power requirements is now available, making its deployment on multiple platforms a possibility (Pollina et al., 2020; Song et al., 2020)
Strategies that have been developed to improve sensor performance include using fluorescence lifetime-based sensing for O2, mechanical wipers for bio-optical measurements, and renewable reagents for CO2 and pH sensors. It would be ideal if more analytes could be detected via time-resolved fluorescence like that available using O2 optodes. Analogous sensors for pH and pCO2 have been extensively tested, but their performance in marine applications has not been promising (Chu et al., 2020). The current trajectory suggests that future in situ sensors will rely heavily on fluidic-based measurement technology.
A near-term opportunity for improving our understanding of Arctic Ocean biogeochemical processes is to more effectively utilize currently available in situ sensors. Combining sensor data with critical physical parameters such as ice thickness or mixed-layer depth can greatly enhance understanding of biogeochemical variability. For example, in a mooring deployment in the Canada Basin (DeGrandpre et al., 2019), pCO2 measurements were combined with upward-looking sonar (ULS) and an acoustic Doppler current profiler (ADCP) to quantify contributions to CO2 variability from ice formation and biological production (based on particle backscatter), respectively. CTD data collected by a nearby ice-tethered profiler were used to estimate mixed-layer depths for computing CO2 mass balances. These types of studies should become more commonplace as interdisciplinary science evolves and grows.
There is also a need for more effective integration of sensors into existing platforms. Currently, only sensors for dissolved O2, nitrate, pH, and bio-optics have been adapted for gliders and autonomous profilers. The platforms can also be improved or newly developed to accommodate larger sensor payloads. Longer-term aspirations could include development of biogeochemical sensors that are deployable on unmanned aerial vehicles (UAVs). Surface ocean profiles of temperature and salinity have been successfully collected using a UAV-deployed dropsonde-microbuoy (Zappa et al., 2020). To do this, sensors would have to be smaller and capable of retaining calibration and functionality after being dropped into the ocean. Only time will tell whether such sensor technology will come to fruition.
Air-Ice-Ocean Boundary Layer Sensors
During MOSAiC (Shupe et al., 2022; Shupe and Rex, 2022, in this issue), the surface energy budget was observed using new, autonomous ASFSs. These mobile, non-floating structures were deployed on existing ice floes alongside ITPs, AOFBs, and IMBs, enabling a complete in situ record of the vertical transfer of heat and momentum in the coupled ocean-ice-atmosphere system. In fully coupled models, sea ice evolution is the result of the simulation of these difficult-to-observe, but highly desirable, heat and momentum budgets, and models are increasingly being used in sea ice forecasting (Smith et al., 2019). In particular, air-ice measurements are rarely made autonomously over sea ice and remain missing from most ITP/AOFB/IMB deployments. Recent advances in higher power, year-long unattended platforms and work to optimize operation of sensors exposed to weather are beginning to address this gap.
In the ocean, the AOFB measures current profiles into the halocline, enhancing the ITP time series, as well as direct eddy-correlation heat, salt, and momentum fluxes, typically at 3 m below the ice interface within the surface mixed layer. Local basal melt rates are measured with a high-resolution altimeter and compared to IMB ice temperature profiles and inferred conductive fluxes. In contrast to equivalent atmospheric sensors, ocean sensors do not typically suffer freeze-ups that greatly compromise sensor performance. ASFSs collect measurements for deriving atmospheric turbulent sensible and latent heat fluxes using eddy covariance methodology and the full radiation budget, and feature conductive flux plates that capture the complete air to ice energy transfer. There are several unique engineering problems with the open-air ASFS platform, including preventing damage from wildlife; managing large-volume, continuous data collection; a need to observe an undisturbed area of the surface adjacent to the buoy hull; and strict tolerances for maintenance of level. Historically, one of the biggest challenges has been mitigating icing on sensors. The fundamental limitation is no longer the arctic-hardening technology, which can be robustly operated (Persson et al., 2018; Cox et al., 2021). Rather, the current challenges are support for such technology with limited power resources afforded by autonomy and considerations for stability and communications on a floating platform that becomes ice bound through natural freezing. For example, two recent iterations of AOFBs were deployed with sonic anemometers but lacked sufficient battery power (by a factor of 100) to keep the sensor unfrozen by heating.
The ASFS proved successful for MOSAiC, but was designed for only two months of autonomy using ~65 W. More recent work in collaboration with the ONR Arctic Mobile Observing System program has reconfigured the ASFS to mount to the freeboard portion of the hull of a large spar buoy designed for multi-year survivability. This platform affords far greater power resources than typical buoys, but nevertheless the redesign needed to provide similar performance on ~75% less power. An experimental deployment of one such system was carried out in autumn 2021, and preliminary indications suggest that mitigating ice on a surface energy budget system is likely achievable in winter in the Beaufort Sea using <20 W. This system also features elements of the AOFB and IMB on the same platform, indicating that there is some progress toward consolidation of the “buoy cluster” onto a common platform. Coordinated deployments with complementary Integrated Arctic Ocean Observing System buoys (clouds and aerosols), unattended lidar buoys (Mariage et al., 2017), and the ASFS/IGB-H are desirable, as is coordination with deployments of autonomous surface vehicles sampling the open water side of the MIZ. In the upper ocean, drifting and mobile platforms described earlier can be used to quantify the horizontal inhomogeneity of surface fluxes, which is critical for understanding the under-ice boundary layer.
Systems of Complementary Platforms
Coordinated deployments of complementary platforms and sensors can provide a more comprehensive characterization of the Arctic environment while placing the measurements in broader spatial and temporal context. Ice-tethered platforms are often deployed in clusters to achieve a more comprehensive set of measurements, such as trios of ITPs, AOFBs, and IMBs. Coordination during planning, deployment, and analysis should be expanded to encompass the growing network of in situ platforms. For example, a joint WARM (Figure 4b,g) and IMB (Figure 4c,d) buoy deployment in 2017 traversed the area where three heavily equipped moorings were deployed (Figure 4a). These moorings, part of the Beaufort Gyre Observing System (Proshutinsky et al., 2020), include a subsurface float (~25 m) with an ADCP and upward-looking sonar for quantifying ice thickness. The moorings also have pCO2, pH, and O2 sensors below the float, and a deep profiling CTD (Figure 4e,f). Most of these in situ biogeochemical studies are not currently coordinated between researchers and organizations. There are, however, international programs to facilitate and inform the community about other studies such as the Global Ocean Acidification Observing Network (GOA-ON). They gather information about observing assets (http://portal.goa-on.org/Explorer) and link to archived data in the National Science Foundation Arctic Data Center (https://arcticdata.io). Additionally, federally funded researchers have access to high spatial resolution commercial satellite imagery from Planet (Planet Team, 2017) and Maxar (https://www.maxar.com), which provide synoptic observations of sea ice conditions surrounding buoy clusters at spatial scales of hundreds of kilometers on near-daily temporal resolution.
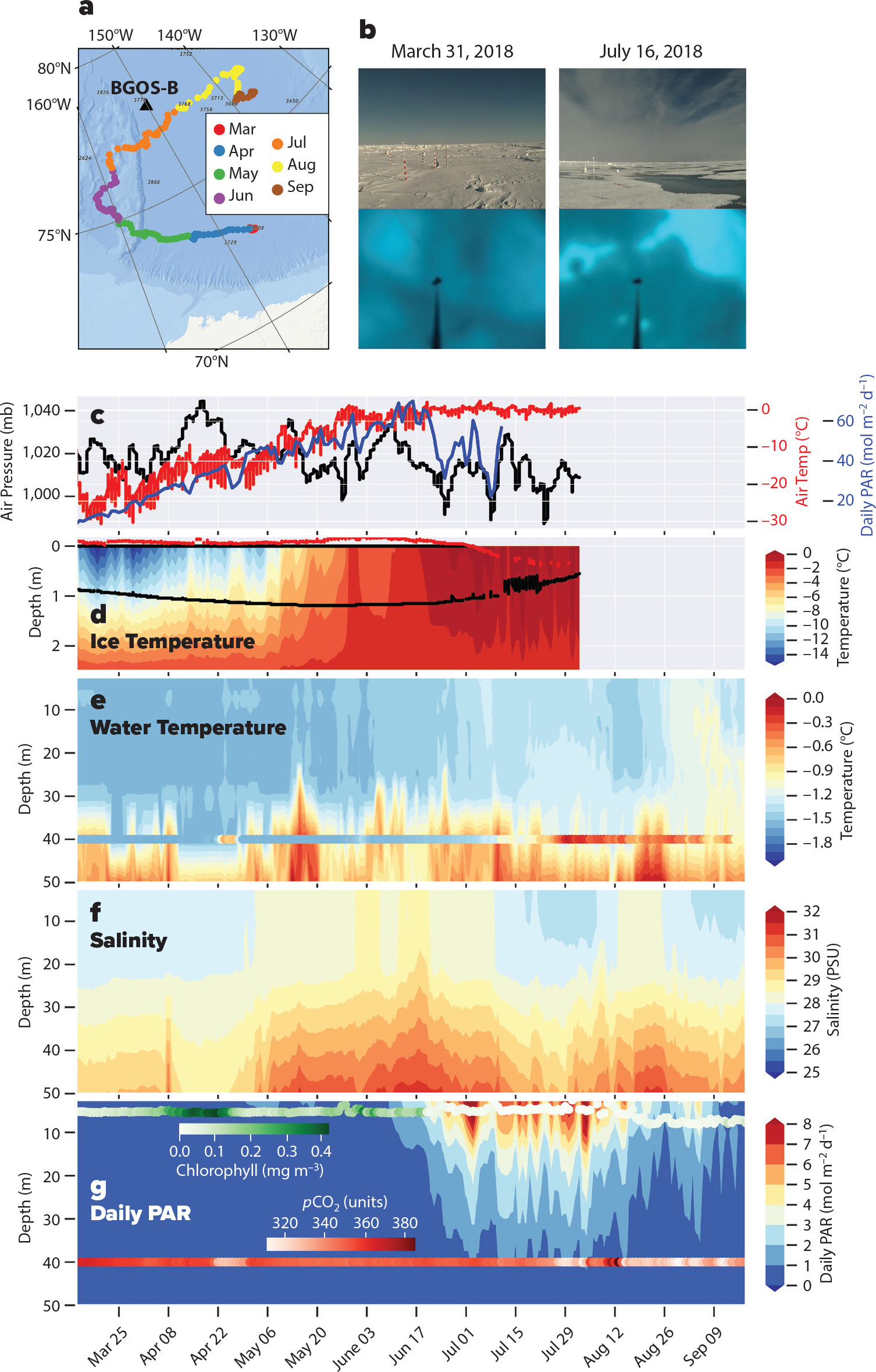
FIGURE 4. (a) Drift track of the WARM buoy and the location of the Beaufort Gyre Observing System (BGOS)-B mooring. (b) Photographs taken from the WARM buoy show views above and below the ice. (c) Air pressure (black) and air temperature both measured by a Sea Ice Mass Balance (SIMB) buoy, along with daily photosynthetically available radiation (PAR) incident on the ice surface measured by the WARM buoy. (d) Ice temperature (contour) and snow surface (red) and ice bottom (black) contours measured by the SIMB. (e) Water temperatures recorded by the WARM buoy with water temperature measured by the mooring overlaid using the same color scheme. (f) Water column salinity measured by the WARM buoy. (g) Daily PAR reaching the water column, chlorophyll fluorescence (green), and pCO2 (red) measured by the WARM buoy. > High res figure
|
Discussion and Recommendations
Autonomous platforms and sensors provide critical capabilities for addressing the severe challenges inherent in Arctic observing. Mature approaches, such as ice-tethered instruments, are being refined and enhanced. Other developments focus on adapting platforms that are in common use in the ice-free oceans (e.g., profiling floats, long-endurance gliders) to overcome the operational constraints imposed by sea ice. Novel sensors provide measurements of critical biogeochemical and biological parameters and the atmosphere-ice-ocean boundary layer. The extended endurance and scalability offered by these instruments makes it possible to resolve key spatial and temporal scales that have previously been impractical or impossible to address. While these advances address the needs of climate/policy and strategic/regional scale systems (Figure 1), meeting the demand for geographically focused, persistent measurements, delivered in near-real time, at short temporal and spatial scales for tactical/situational awareness (Figure 1, lower left quadrant) remains challenging.
Continued progress toward meeting the spectrum of Arctic observing needs will require highly scalable instruments that are serviceable with modest, flexible logistics. Effort should be focused on development of low-cost, lightweight platforms and sensors that support a range of operational modalities, with the potential for deployment in large numbers. Such flexible systems could be applied to problems ranging from process studies to climate-scale observing and could adapt in response to changes in the Arctic environment, societal needs, and scientific understanding (Figure 1). The most effective observing systems are likely to be designed using heterogeneous combinations of complementary platforms. Robust, low-cost, operationally simple platforms open a path for regional applications through collaboration with coastal communities, thus broadening the range of people able to assist in the design and maintenance of future observing systems. These developments would also enable opportunistic deployments in more remote regions of the Arctic through agreements with vessels involved in both commerce and tourism as ship traffic increases in the future.
Additional technological priorities should focus in areas with broad impact. Accelerated development of sensors capable of collecting long-term measurements of surface energy budget components and of biogeochemical and biological parameters, ideally at scales similar to those resolved for physical variables, should be prioritized. Underwater geolocation at basin scales, such as that provided by nascent very low frequency acoustic systems, would facilitate operation of a broad range of low-cost autonomous platforms for operation in ice-covered regions. Data exfiltration from instruments operating under the ice remains extremely challenging, but progress in this area is critical for systems meant to support operational modeling.
Measurements must be distributed over the entire Arctic Ocean, including the poorly sampled Russian sector, to take full advantage of the complementary nature of remote sensing and in situ observations. Remoteness makes surveys and instrument deployments difficult. Cyclonic circulation in the Russian Arctic drives divergent drift patterns that rapidly expel instruments from the region. In the future, autonomous profiling floats and gliders may extend our measurements into these remote regions. Another promising approach is increased use of long-range aircraft for float and buoy deployment, perhaps in conjunction with airborne hydrographic surveys using expendable probes (Dewey et al., 2017; Zappa et al., 2020). This suggests investing in efforts to adapt instrument systems described in this section to aircraft deployment over wider areas and more seasons.
Acknowledgments
We thank two anonymous reviewers and guest editor Tom Weingartner for their careful reading and for helpful, constructive suggestions that strengthened this paper. Arctic observing efforts have benefited from sustained support from resource sponsors that include the National Science Foundation Arctic and Arctic Observing Network programs, the Office of Naval Research Arctic and Global Prediction program, the National Oceanic and Atmospheric Administration, and the National Aeronautics and Space Administration. For the preparation of this paper, CML was supported by ONR grant N00014-19-C-2076 and NSF grant OPP-1902595; MDD by NSF OPP-1951294; JG by OPP-1842306; VH by NSF OPP 1603548; RK by NASA grant 80NSSC22K0392; JM by OPP-1842306; CJC by NOAA’s Global Ocean Monitoring and Observing (GOMO) program and Arctic Research Program (ARP); HS by NSF grants OPP-0425838, EEC-9986821, and NASA grant Z601701; and TS by ONR grant N00014-20-S-B001 and NSF grant OPP 1723400.