Introduction
Habitability is broadly defined as an environmental state with liquid water, chemical disequilibria, and the elemental and organic molecules needed for life as we know it. In addition to having the basic ingredients for life, habitable environments must also exist within the pressure, temperature, pH, and water activity boundaries that allow cells to persist (Dartnell, 2011). Could life as we know it survive in liquid water environments elsewhere in the solar system? A practical strategy for assessing habitability quantitatively is to determine the physicochemical conditions that would make life possible on extraterrestrial ocean worlds. (Hereafter, “ocean” refers to Earth’s ocean and “ocean worlds” refers to oceans on other extraterrestrial bodies.) These conditions include the availability of water, sufficient nutrients (e.g., C, N, P, S, Fe) in bioavailable forms, and energy that could be used by life. In most cases, these conditions are completely unconstrained by present observational data because it is difficult to access subsurface liquid water environments beyond Earth. However, as exploration of icy ocean worlds matures from initial reconnaissance to more in-depth characterization, opportunities will arise to obtain critical information about physicochemical conditions of ocean worlds (Hand et al., 2020). Near-term opportunities may involve serendipity, such as plume eruptions from the ocean on Saturn’s moon Enceladus, or less direct sources of information, such as the compositions of planetary surfaces or atmospheres of ocean worlds (for an overview, see Glein and Zolotov, 2020).
What do we know about the ocean habitability of Earth, Europa, and Enceladus?
Earth
Earth is the only planetary body in the universe that we know for certain is inhabited. Every drop of water in our ocean is potentially habitable and likely inhabited by a diverse array of life. This habitability is due to multiple factors: critical nutrients are recycled through ocean circulation, pH is buffered by Earth’s carbonate system, and clement temperatures and pressures are maintained largely due to Earth’s atmosphere, to name a few (Langmuir and Broecker, 2012). Ocean circulation exerts a fundamental control on Earth’s ocean habitability at a variety of spatial scales and is critical for moving heat and dissolved gases, as well as nutrients needed for life, throughout the ocean (Siedler et al., 2013). Ultimately, the habitability of Earth’s ocean also depends on plate tectonics, which creates new oceanic crust that provides fresh substrates for life (Korenaga, 2012) and results in water-rock reactions that lead to the discharge of nutrient-rich waters at hydrothermal vents and at other seafloor locations (Govenar, 2012). Subsequent rock weathering releases critical nutrients, such as phosphorus, that would otherwise limit the growth of marine life.
Europa
The possibility for developing a biosphere on ice-capped ocean worlds with limited sunlight, such as Jupiter’s moon Europa, likely depends on the upward movement of reduced chemicals from the moon’s silicate interior to its ocean via water-rock reactions at the ocean floor (Vance et al., 2016) and the downward movement of oxidants (e.g., H2O2; Carlson et al., 1999) via the ice shell into the liquid ocean from the irradiated surface (see Schmidt, 2020, for a review). This exchange is highly likely on Europa and could provide energy to support a dark biosphere (Chyba, 2000; Chyba and Phillips, 2001; Hand et al., 2009). Russell et al. (2017) argue that areas of relatively high oxidant fluxes at the icy lid of a Europan ocean should be expected to be highly biologically productive. The details of how these fluxes may occur are currently unknown, but evidence for global tectonism (Pappalardo et al., 1998; Doggett et al., 2009; Kattenhorn and Prockter, 2014) and recent cryovolcanic activity (Schmidt et al., 2011; Roth et al., 2014; Sparks et al., 2017; Jia et al., 2018) suggests that current ice-ocean exchange is possible via ice shell processes on Europa. With tidally induced magmatic activity providing global energy to Europa, it is possible that the conditions to support life have existed on this moon over the history of the solar system (Běhounková et al., 2021).
Enceladus
Exploration of Enceladus by NASA’s Cassini mission provided important lessons on how we can investigate the habitability of an extraterrestrial ocean world (Cable et al., 2021). As an example, Cassini found hydrogen gas (H2) in a plume that emanates from fractures in the south polar terrain. The likely source of H2 is ferrous iron oxidation via water-rock reactions that occur below Enceladus’ seafloor (Waite et al., 2017). Using a combination of mass spectrometry measurements in the plume and geochemical modeling of carbonate equilibria, researchers constrained the pH of Enceladus’ ocean to ~9 (Glein et al., 2015; Glein and Waite, 2020). Enceladus also taught us that hydrothermal processes (Hsu et al., 2015) can be a critical component of energy generation on ocean worlds beyond Earth (Choblet et al., 2017). The abundance of H2 was found to be sufficiently high for methanogenesis to be energetically feasible (Waite et al., 2017; Taubner et al., 2018). A recent statistical study by Affholder et al. (2021) suggests that methanogens may be the most probable source of observed methane if the origin of life is not an improbable event there. Surface-derived oxidants and oxidants produced as a result of decay of dissolved radioactive 40K within the ocean could also contribute to supplies of chemical energy in Enceladus’ ocean but to a lesser extent than does methanogenesis (Ray et al., 2021).
To what extent can Earth’s ecosystems serve as analogs for other ocean worlds?
Unlike all other ocean worlds in our solar system, most of Earth’s surface ocean is in direct contact with the atmosphere and is bathed in sunlight. Although some organisms live off the chemical energy and minerals supplied by oceanic hydrothermal vents (called chemosynthesis; Dick, 2019) and live within the fluid-filled pores of crustal rocks (Li et al., 2020), Earth’s ocean productivity is largely driven by photosynthesis in the surface layer—the euphotic zone—where sunlight penetrates. On ice-capped ocean worlds, sunlight may not penetrate the ice sufficiently to reach the liquid ocean, and wind-driven ocean mixing would be absent. More relevant Earth analogs for habitable environments on other ocean worlds are interfaces, such as ice-water and seafloor-water boundaries (e.g., hydrothermal systems). Other analogs include habitats inside of ice, snow, ocean crustal rock, and clathrates (compounds formed by the inclusion of molecules of one kind in cavities of the crystal lattice of another).
Interfaces
Some of the most productive and diverse aquatic habitats on Earth are found along boundaries and interfaces where biomass is concentrated. Examples include the seabed (sedimentary and igneous) and the underside of floating ice. Large-scale ocean processes deliver energy, chemicals, and organisms to these boundaries, which obstruct further transport, thereby concentrating life and life’s necessities, and enabling biology to proceed more vigorously than in more dilute seawater. Interactions at the interfaces that control atmospheric deposition, exchanges across the coastal zone, and ice dynamics often control elemental cycles. On Earth, these interfaces are areas where marked changes in the physical, chemical, or geological structure of marine, brackish, and freshwater environments take place continuously. Growth rates of organisms in these habitats often change rapidly, from day to day, and over short distances.
Some of the most relevant Earth analogs to other ocean worlds are the unique habitats formed at boundaries between ice and water. Sea ice plays a dual role in the control of high-latitude phytoplankton blooms by influencing both light shading and stratification (Arrigo et al., 2012; Kauko et al., 2017). Melting of ice in the spring can stabilize the water column, supporting large phytoplankton blooms. The timing, size, and type of ice edge algal blooms can define the success of a growth season and control food web dynamics (Ducklow et al., 2013). Phytoplankton blooms can occur with limited light under several-meter-thick layers of ice and snow, as studied under Arctic sea ice (Boles et al., 2020) and in Antarctic dry valley lakes such as Lake Bonney (Obryk et al., 2014), Lake Fryxell (McKnight et al., 2000), and Lake Vanda (Sumner et al., 2016). Limits to phytoplankton growth under thicker ice layers are not well known. Certainly, heterotrophic and mixotrophic microbial life can also be important along boundary habitats, given nutrient availability in the form of dissolved organic matter. Even without penetration of direct sunlight, physiological adaptations of microorganisms to ocean-ice dynamics could serve as analogs for adaptations of both autotrophic and heterotrophic life in other icy ocean worlds.
The undersides of floating Antarctic ice shelves vividly illustrate the importance and roles of interfaces. Murray et al. (2016) discovered a vibrant, diverse ecosystem, including abundant sea anemones, at the undersurface of the floating Ross Ice Shelf. A similar community observed under Thwaites Glacier (Schmidt, 2020) shows the possibility for dense, complex, and energy-intensive biological activity in an unexpected and astrobiologically highly relevant location. Because the icy shells also form and evolve from cyclic freezing and melting, analogous processes to ocean-derived ices on Earth are helping to constrain the active cycles within the ice and at its interfaces (Buffo et al., 2018, 2019) as well as cycles of water within the ice shell (Kalousová et al., 2014; Chivers et al., 2021).
Life is abundant at the seafloor-ocean interface. There, unusual microbial and invertebrate populations survive by feeding on organic material made from CO2 reduction by chemotrophic bacteria that oxidize inorganic compounds released by hydrothermal vents (Van Dover and Trask, 2000). Life persists beneath Earth’s seafloor in low-energy environments within ocean crustal rocks and sediments (Jones et al., 2018). The seafloor-ocean interface is where photosynthesis is postulated to have begun under low-intensity, long-wavelength geothermal light (Martin et al., 2018). Anaerobic green sulfur bacteria, for example, are capable of photosynthetic growth at extremely low light intensities. Temporally variable light has also been observed in visible wavelengths (400–600 nm) at deep-sea vents. This lighting is orders of magnitude greater than predicted for a thermal source, and the enhancement is likely caused by mechanisms associated with turbulence, mixing, and precipitation, such as vapor bubble luminescence, chemiluminescence, crystalloluminescence, and triboluminescence (White et al., 2002). Studies of marine microalgae in the polar night can also contribute to our understanding of low light photosynthesis, and the polar night can serve as an analog of the light conditions in the deep sea (Cohen et al., 2020; Johnsen et al., 2020) and potentially on icy ocean worlds.
Earth analogs for habitable regions on ocean worlds may also exist on land, particularly in polar regions. One fascinating example is found at the upper interfaces, or “lids,” of Antarctic subglacial lakes, where overlying glacial ice meets subglacial lake waters. Molecular oxygen is supplied to the lids of some subglacial lakes during melting of air-clathrate-bearing ice. Air is trapped by progressive burial under new snow at ice sheet surfaces. At depth, the air is forced into cage-like structures in the ice crystal lattice under high pressure; the ice flow then carries such clathrates over subglacial lakes. Although oxygen in this case is photosynthetically derived, it would seem likely to play a similar role in dark, chemosynthetic environments for surface-derived oxidants in the Europan ice shell (Hand et al., 2006). The ice accreting above these lakes is also among the lowest energy niches on Earth, which provides an important lower limit on both the energy required to sustain and the technology needed to detect life in ocean worlds (Hand, 2017). Spatial variation in oxygen delivery within and between lakes (Winebrenner et al., 2019) is likely to result in a variety of habitable niche space (Mikucki et al., 2016). In some subglacial lakes, electrons derived from rock-water interactions, or the flux of reduced geothermal fluids, support oxidation-reduction reactions that drive a suite of biogeochemical cycles (Livingstone et al., 2022) providing a diversity of natural laboratories to study life under ice.
Life Inside Ice
On Earth, there are active habitats within ice, as well as at its outer boundaries. It is presumably the case that a habitable ocean world would also have habitable niches within the ice shell. On Earth, this means that research into the processes that occur within ocean-formed ice—sea ice—and the cycling and processes that define their ecology, are relevant to ocean worlds and their processes as well (Buffo et al., 2018, 2019; Schmidt, 2020). On the biological side, snowpacks serve as habitats and nutrient reservoirs for microbes across the global cryosphere on all continents (Lutz et al., 2016; Hodson et al., 2017). Snow or glacial algae survive in a liquid water film between melting snow and ice crystal and may color snow green, golden-brown, red, pink, orange, or purple-gray (Hoham and Remias, 2020). Formation of snow algae blooms is related to light availability, liquid water content, and temperature (Hoham and Remias, 2020). Algal pigments can be photoprotective and shield snow algae from harsh light conditions (Bidigare et al., 1993). They can also play a functional role in changing the albedo of snow, causing snow and ice to melt and creating more of the liquid water required for algal growth (Dial et al., 2018). With some exceptions, snow algae are usually found in snow with acidic pH. Snow and ice algae can be ephemeral, active only on the order of weeks during spring and summer when air temperatures remain above freezing, and on small spatial scales, often in the borders of ice and land margins. Nutrient cycling can be locally diverse and include volcanic sources, leaching from underlying rock, marine fauna (e.g., penguin and seal excreta), as well as intense cycling within glacial microbial communities.
In addition to the air clathrates described above, methane hydrates may be the most accessible habitats in which to find life on other planetary bodies in our solar system. Beneath the seafloor of Earth’s continental margins, sediments associated with methane clathrates host unique microbial assemblages (Inagaki et al., 2006; Glass et al, 2021), and these microbes encode proteins capable of altering clathrate properties (Johnson et al, 2020). Gas hydrates are likely present on other planetary bodies in our solar system (Mousis et al., 2015) and may play a role in creating and sustaining liquid oceans, especially on ocean worlds without active sources of tidal heat (Tobie et al., 2006; Kamata et al., 2019). At the Martian poles, methane clathrates could be present at depths as shallow as only half of a meter below the surface and extend down several dozen kilometers (Gloesener et al., 2020). These habitats have potential for serving as pockets of liquid water for microbial refugia in the deep subsurface and may be accessible to future drilling missions. On the seafloor above gas hydrates, methane escaping from the seafloor serves as an energy source for extensive communities of microbes and the tubeworms and clams that feed on them at cold seeps (Levin et al., 2016).
What technology and integrative scientific approaches can enable us to probe the habitability of currently inaccessible oceans?
Organics
The next addition to our toolbox for quantifying habitability is organic molecules. Conventionally, organic molecules are targeted as potential biosignatures (Neveu et al., 2018) or precursors to life. However, observations of outer solar system bodies suggest that abiotic organic compounds may be abundant planetary materials, possibly of comparable importance to water and rocks (Néri et al., 2020). This means that organic compounds could play a major role in planetary evolutionary processes, such as hydrothermal alteration (Postberg et al., 2018). Geochemical experiments show that many groups of organic compounds behave predictably in hydrothermal systems, where abundant organic compounds are driven toward states of metastable equilibrium, defined by the physicochemical conditions of the system (Seewald, 1994; Shock et al., 2019; Robinson et al., 2020). These relationships provide the potential to derive properties (e.g., temperature, oxidation state, pH) of the host hydrothermal system from measurements of organic compounds. However, fully realizing the power of this approach and understanding its limitations will require (1) improvements in our ability to quantify the thermodynamic properties of organic compounds at high temperatures and pressures, (2) additional experiments to determine the mechanisms and corresponding rates of organic reactions under icy ocean world conditions (e.g., in carbonate/ammonia-rich aqueous solutions and in the presence of primary and secondary minerals commonly found in chondritic bodies), (3) development of models that track the coupled evolution of diverse water-rock-organic compositions during hydrothermal circulation and other transport processes, and (4) ground-truth field tests of the prevalence of metastable equilibria among different compound classes at actively serpentinizing systems (e.g., Lost City Hydrothermal Field in the Atlantic), where organic compounds can be synthesized abiotically, and at organic-rich hydrothermal systems such as Guaymas Basin, which is a potential analog for hydrothermal processing of organic matter on worlds that have accreted an abundant organic component.
Autonomous Platforms for Interface Exploration
Interfaces are critical areas where physical, chemical, and biological processes act on a variety of spatial and temporal scales. Intense biological activity, accumulation of organic and inorganic substances, and unique interactions that deviate from general conditions occur at these locations and can form an environment that may be extremely favorable for life. Understanding exchange and movement at interfaces of nutrients, gases, heat, and redox potential and quantifying light production and availability in low light environments such as deep-sea vents and the polar night are essential to quantifying habitability of oceans and to guiding searches toward the most habitable environments. Environmental processes along interfaces typically have shorter temporal scales (hourly) and spatial scales (meters to kilometers) and often require different methods for assessing change compared to other ocean habitats.
Study of ice-ocean interactions is an expanding area in the research into ocean worlds, and it is growing in fidelity. Active research by the climate and polar research communities regarding the fundamental controls on the circulation of oceans under ice can benefit this work (Holland and Jenkins, 1999; Dinniman et al., 2016; Holland et al., 2020). Such understanding hinges on ocean modeling on large and intermediate scales, as well as coordinated, focused observations of fluxes and processes near the interfaces. Already, some modeling of ocean world circulation exists (for a review, see Soderlund et al., 2020). However, the details of salinity, nutrient, and putative biomass distribution by ocean circulation is currently not included, because fundamental processes are still being resolved, such as overturning circulation, ice pumping, or double diffusion. Laboratory research in cyclic freezing and melting should be expanded in order to study the effects of freezing as a means of changing the composition of reservoirs and the ice, as well as to understand the fundamental sources of chemical energy possible in these environments.
Technology development is essential both for accessing interfaces in challenging places (e.g., beneath ice shelves, at the lids of subglacial lakes, and on and in the deep seafloor) and for measurements to diagnose poorly known phenomena occurring there (e.g., heat, salt, light, and nutrient fluxes beneath the ice shelves). New in-water autonomous platforms with a variety of sensors are being deployed to investigate the biodiversity and the time-dependent behavior of under-ice blooms (Kukulya et al., 2016; Hill et al., 2018; Johnsen et al., 2018; Randelhoff et al., 2020; Lawrence et al., in press; see Figure 1a–f for examples). Data collected by these platforms are especially important for understanding the dynamics of life associated with the underside of ice during winter and spring when it is challenging to assess with many remote-sensing techniques. New technology can motivate a variety of new scientific questions, for example, on interface processes, and spur further technology development for more extensive exploration of this habitat (Rack et al., 2012). Similarities between Earth’s ocean-ice interactions and those that likely also operate on ocean worlds (with important implications for geochemical cycling and climate response of ice shelves) means that exploring these physical processes on Earth provides feed-forward context for studying other ocean worlds. Exploring these sea ice-covered regions on Earth using ice-penetrating radar, seismic methods, and underwater vehicles is often (but not always) technologically synergistic with needed developments for exploring ocean worlds. The upcoming NASA Europa Clipper mission (expected to launch in 2024) will provide in situ plume/atmospheric and remote-sensing measurements of the composition and detailed local context of ice-ocean exchange processes that was not possible with earlier missions (Howell and Pappalardo, 2020). How these processes interact with more regional-scale geologic processes on bodies like Enceladus is an area of active research (Walker and Schmidt, 2015; Běhounková et al., 2017; Nimmo et al., 2018; Weller et al., 2019; Kang and Flierl, 2020).
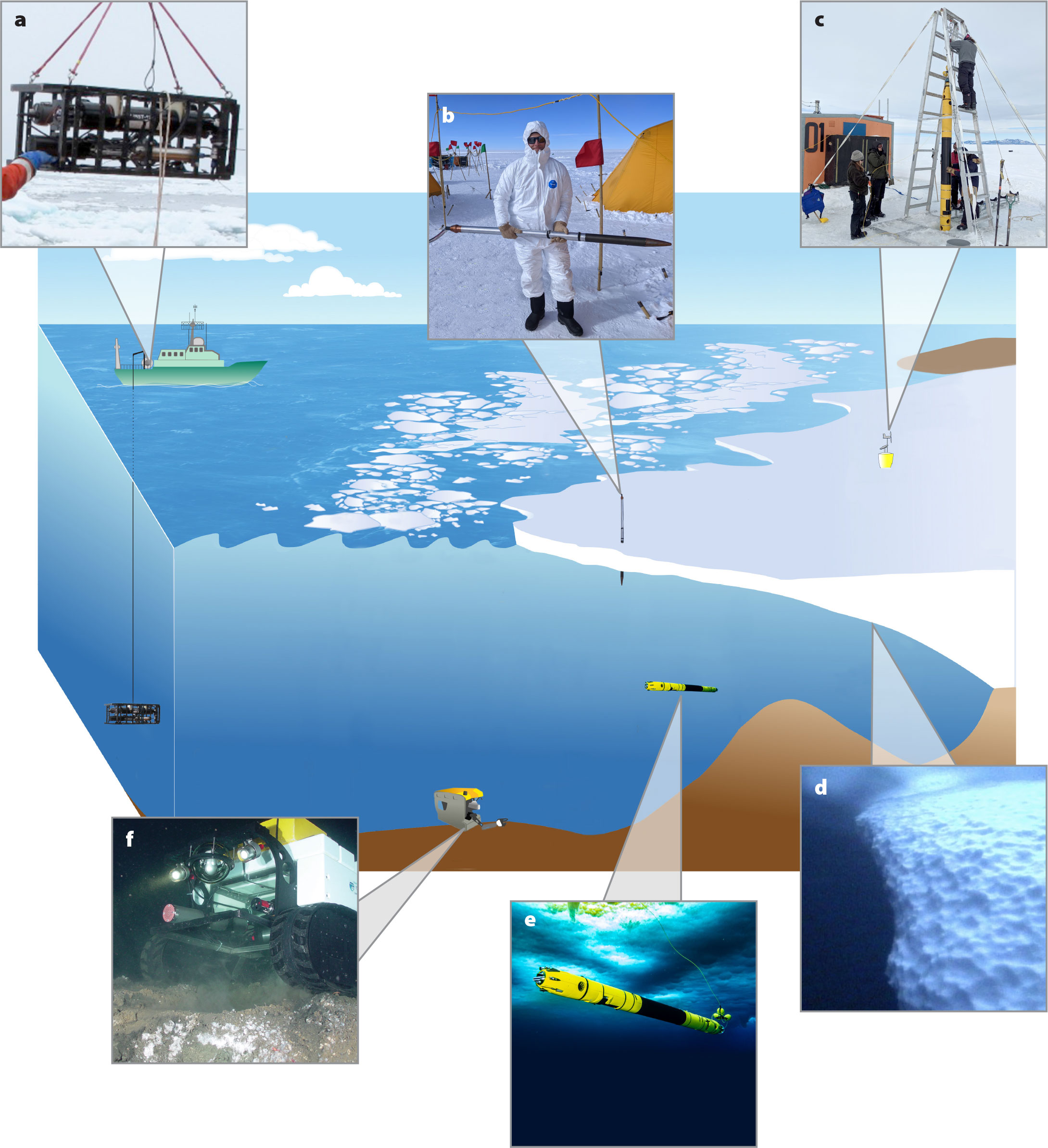
Figure 1. Examples of instrumentation for ocean world analog exploration. Background figure modified from Newman et al. (2019) (a) Optical sensors are deployed during the ORCAS/LTER field campaign in 2016 in the Southern Ocean. Image credit: Shungudzemwoyo Garaba (b) A researcher holds a University of Washington Ice Diver thermal melt probe during field testing at Greenland Summit in May 2021. The Ice Diver is in development to access deep Antarctic subglacial lakes (>100 m depth beneath the ice surface) at logistical costs much lower than those of conventional drilling. Field testing demonstrated microbiologically clean sampling using peristaltic pumps and autoclaved tubing and sample bags (German et al., 2021; Caleb Schuler, University of Tennessee, pers. comm., 2022). (c) Icefin, a modular underwater vehicle designed to explore Earth’s polar oceans in ways that inform future planetary exploration (e.g., Meister et al. 2018), is deployed using a launch frame and lift system to raise the vehicle to vertical for deployment through narrow (~30 cm) boreholes through sea ice or ice shelves. Image credit: Icefin/RISEUP/Anthony Spears (d) This underwater image of melting of the face of the Erebus Glacier Tongue, Antarctica, was taken by Icefin in 2018. The cusps on the surface are caused by melting of the ice in turbulent water. Image credit: Icefin/RISEUP/Britney Schmidt (e) Icefin, viewed from underwater, conducts a mission below ~2 m of sea ice cover in McMurdo Sound. Icefin carries over 10 science sensors and can also map ocean currents, nutrients, pH, and the seafloor. Image credit: Rob Robbins (f) Cabled benthic crawler Wally collects data for studies of gas hydrate outcrops on the seafloor. For more information, see Reidel et al. (2022). Image credit: NEPTUNE Canada. > High res figure
|
Modeling
Resolving the phenology of diverse life forms is crucial for understanding responses to environmental perturbations but is impossible to capture with a single snapshot. Investigations require careful time series of measurements and modeling across relevant timescales. To achieve this, it will be critical to measure and model the patterns and dynamics (spatial and temporal) of ice and oceans on both this planet and other ocean worlds. Using Earth as analog, we can begin to identify essential model parameters that are needed to improve ocean, ice, and crustal models, and evaluate new and existing technologies and models relevant to studying ocean, ice, and crustal dynamics of other ocean worlds.
Biogeochemical and ecosystem models are advancing our ability to characterize the complexity of life in our own ocean world of Earth. For example, marine biogeochemical and ecosystem models include parameters for the physical environment (ocean temperature, light, salinity, and circulation), the cycling of inorganic and detrital matter (biogeochemistry), and the explicit representation of some portion of the living component of the ocean (e.g., phytoplankton, zooplankton) (IOCCG, 2020). Such integrated modeling tools could be adapted for assessing the parameters and potential for life in other ocean worlds (Farrell et al., 2021). Advances are being made in measuring and modeling mesoscale and submesoscale circulation patterns (e.g., eddies, streamers, and fronts) and their roles in transporting heat and nutrients into and out of the euphotic zone (McGillicuddy, 2016).
The general approach of using modeling to help fill in the gaps where data are incomplete provides a tool for extracting maximal information on extraterrestrial ocean properties. This type of tool was not developed until late in the Cassini mission, which was launched in 1997 and ended in 2017. Future ocean worlds missions should plan integrative approaches from the start, during the development/testing of instruments and planning of mission operations, and these approaches should include full traceability that links primary mission data to ocean properties for a broad range of models (e.g., MacKenzie et al., 2021). More generally, a key science lesson is that ocean habitability involves other parts of the planetary body dynamically interacting with the ocean. This echoes our understanding of the Earth system and suggests that coordinated geophysical and geochemical studies are needed to better understand how exchange processes can create habitable conditions on ocean worlds. Modeling seawater chemistry and water-rock interactions based on analogous processes on Earth, extended to hypersaline lakes and deep anoxic hypersaline basins with more exotic chemistries (Fisher et al., 2021; Buffo et al., 2022), would benefit both the Earth and the astrobiology communities.
To better understand the habitability of gas-hydrate-bearing environments, it will be necessary to measure and model the patterns and spatial and temporal dynamics of methane clathrates, determine the essential model parameters necessary to improve gas hydrate models, evaluate new and existing technologies and models relevant to studying hydrate dynamics, and pursue better characterization of microbes in their native hydrate habitats on Earth.
Interdisciplinary Efforts
Coordination between the Earth/ocean sciences and the planetary science communities will be critical for next generation studies of ocean world habitability, and—ultimately—life detection (NASEM, 2022). Earth/ocean scientists have a deep base of experience on how life can be linked to environmental conditions and processes, while planetary scientists can translate the underlying concepts to alien environments and indirect spacecraft measurements. It is important to connect the understandings of various layers of a planet/moon in order to plan effective observations because the ice shell and atmosphere are the windows through which we will, for now, observe ocean world systems. How does the ice reflect or hide the ocean? How do seafloor heating and rotation drive ocean currents, and how do they distribute biosignatures, heat, salt, and other components? Is the ice-ocean interface the first habitable niche, or are there locations in the ice or along the seafloor to search? Synergy between observations of ice, ocean, and geothermal processes on Earth and models of how these processes may manifest on ocean worlds benefits both the Earth/ocean sciences and the planetary science communities. Moreover, the technological needs for exploring, especially at the poles, is often synergistic. Terrestrial and extraterrestrial ocean scientists must work collaboratively to measure and model spatial and temporal dynamics, determine essential parameters that govern interface processes, and evaluate new and existing technologies to access and study dynamics of habitable worlds.
Acknowledgments
The idea for this manuscript came out of a December 2019 joint NASA-NSF workshop “Oceans Across the Solar System” co-chaired by Laura Lorenzoni and Mary Voytek. We thank Jill Mikucki for contributions to this manuscript and Ashley Kleinman for assistance in preparing it for publication. Funding to JBG and BES was provided by the Oceans Across Space and Time (OAST) project, funded by NASA’s Astrobiology Program award 80NSSC18K1301. Funding for BES was also provided by SESAME grant 80NSSC19K0615, and Icefin work was provided by NASA PSTAR program RISE UP NNX16AL07G and NSF-NERC ITGC Thwaites program #1739003. Funding for HMD was provided by NASA’s Ocean Biology and Biogeochemistry program (80NSSC20K1518). Funding for CRG was provided by NASA ICEE2 grant 80NSSC19K0611. Funding for DPW was provided by NASA SESAME grant NNN12AA01C with prior sampling and jetting melt probe development supported by NASA COLDTECH grant NNX17AF68G.