INTRODUCTION
The search for biological life in icy worlds on Earth and on planets and their moons requires an understanding of key physical, chemical, biological, and geological processes (Hendrix et al., 2019). There are strong synergies between the exploration of icy moons and studies of polar oceans, ice shelves, and ice-ocean interfaces on Earth that will allow us to expand our capabilities for investigating and understanding such environments throughout the solar system (Hendrix et al., 2019). In both the Arctic and Antarctic (Figure 1), ongoing environmental changes are modifying physical and biological systems (Frey et al., 2021; Meier et al., 2021; Moon et al., 2021). Due to its longstanding research support infrastructure, the Antarctic has specific sites that can provide access to subglacial lakes that are overlain by multiple kilometers of ice (German et al., 2022, and Glass et al., 2022, both in this issue). There are also opportunities to study ice-ocean interface conditions at polar ice shelves that are underlain by a subsurface ocean, such as beneath the Ronne Ice Shelf in Antarctica (Hatterman et al., 2021) and the Devon Ice Cap in the Arctic (Rutishauser et al., 2018). Both have been subject to variable basal melt due to warming waters associated with climate change. The production of high saline pools of water below ice shelves has implications for understanding key processes in icy world sites (Hendrix et al., 2019).
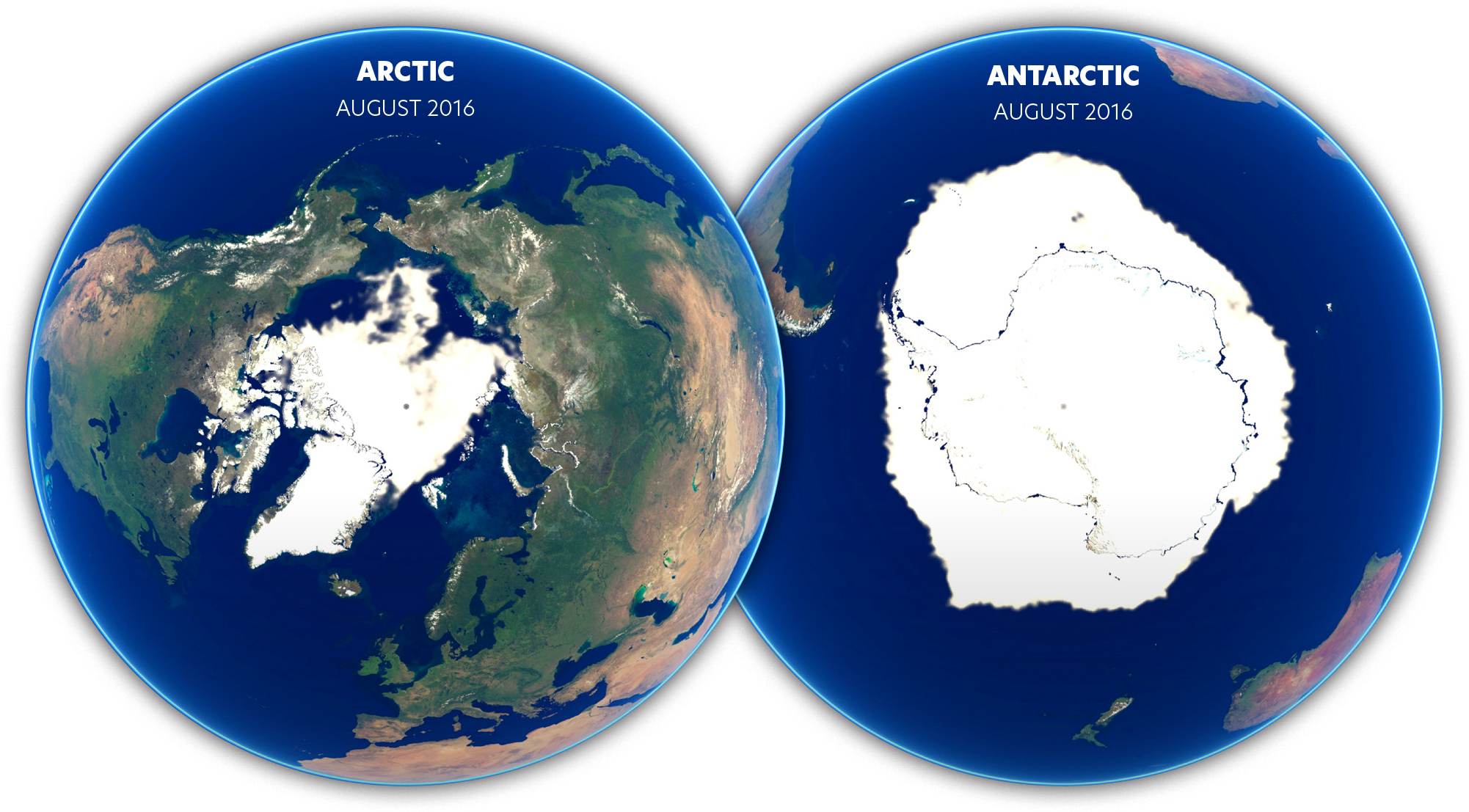
|
Exploration of Earth’s deep ocean is essential to the search for life in the solar system. Hydrothermal vents are key areas for cross-linked Earth and planetary studies relevant to deep ocean dynamics and local biological components. Microbes can use reduced compounds released at vent systems as energy sources and are themselves then consumed by multicellular life forms (e.g., tubeworms and clams; Hand and German, 2018; Hendrix et al., 2019).
The oceans of Jupiter’s moon Europa and Saturn’s moons Enceladus and Titan are key targets in the planetary search for biological life forms in the solar system. However, much of Titan’s icy outer shell is too thick (up to 200 km) for robotic exploration. By contrast, Europa and Enceladus have icy shells that are several tens of kilometers thick and therefore constitute potentially explorable environments (Howell and Pappalardo, 2020). Enceladus and Europa most likely have deep global oceans that are in direct contact with rocky interiors at their greatest depth. As an example, there is strong potential for finding liquid water on Europa, whose icy shell ranges from perhaps 10 km to 40 km thick, overlying an ocean up to 130 km in depth that covers a rocky core (Figure 2; Howell and Pappalardo, 2020). The icy shell (and potentially the rocky interior) flexes in response to gravitational interactions with Jupiter at multiple tidal frequencies in a day (Thomson and Delaney, 2001). This motion can produce heat that may support mechanisms necessary for producing a liquid ocean with chemical components as well as facilitating ocean circulation. If true, there is a high potential for water-rock reactions and chemical exchanges like those that occur at hydrothermal vents on Earth (Van Dover and Trask, 2000) and that could provide chemical energy to support life. With ongoing geological activity due to the gravitational interactions with the host planet (German et al., 2022, in this issue, and references therein), transport within the fluid ocean might include chemicals released at both the ice-ocean and rock-ocean interfaces (Kang et al., 2022). Such interactions occur in Earth’s polar regions and at deep-sea hydrothermal vents, respectively. Connecting Earth-focused oceanographic and planetary scientists in joint studies could benefit both groups. In addition, ongoing ice-focused studies on the hydrosphere and cryosphere by glaciologists and geophysicists are important within this collaborative framework.
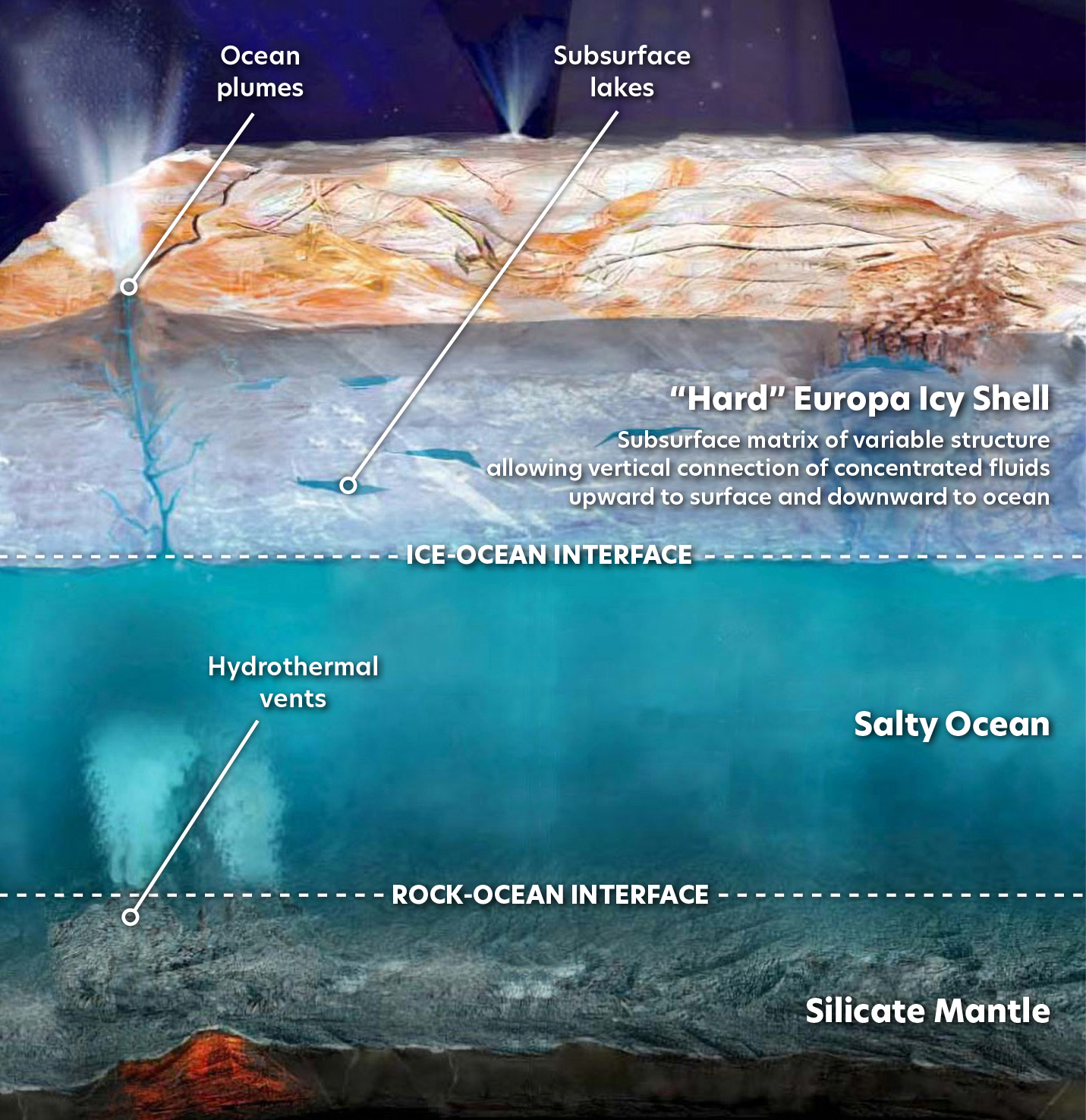
FIGURE 2. Artistic representation of Europa, an icy moon of planet Jupiter, in cross section. Labels show key interfaces and potential mechanisms described in the text regarding icy shell dynamics of Europa as interpreted from Earth studies. Hydrothermal vents release minerals to the salty ocean above at the rock-ocean interface. The cold, surface icy ocean has a variable matrix that allows venting of ocean water to the atmosphere as well as potentially downward release of concentrated, entrained ocean fluids to below the icy shell. If these occur, there is a potential for subsurface pools or lakes of reduced chemicals. These subsurface lakes and hydrothermal vents might support microbial life, similar to systems on Earth. Image modified from Hand et al. (2017). > High res figure
|
OCEAN WORLDS WORKSHOP GOALS, QUESTIONS, AND SUMMARY
In December 2019, NASA held a workshop in California entitled “Ocean Science Across the Solar System,” with a goal of developing collaborative topics for joint Earth and planetary science investigations. Below are three questions discussed during the workshop related to climate change, along with topical summaries.
1. How can studies focused on climate change on Earth provide a better understanding of the evolution of an ocean world?
Climate change is impacting Earth, including its regional weather patterns, ocean dynamics, and geology, processes that are relevant to understanding the planetary dynamics of ocean worlds (Nimmo and Pappalardo, 2016; Hendrix et al., 2019; German et al., 2022, in this issue, and references therein). Earth’s cryosphere modulates the global climate system through atmosphere-ice-ocean interactions (Meredith et al., 2019, and references therein). Changes in sea ice extent and thickness, melting freshwater glaciers, and variable ice sheet dynamics are all responding to a changing climate. Mechanisms for seasonal ice growth and dissolution can directly impact regional weather patterns and global ocean circulation (Lumpkin and Speer, 2007). Ongoing studies of these oceanographic and cryospheric processes in Earth’s polar regions are directly relevant to gaining a better understanding of the mechanisms of ice-ocean interactions in planetary studies (Hoehler et al., 2022, in this issue).
For example, formation of frazil ice and congelation (sub-ice) growth beneath ice shelves are relevant to studies of icy worlds (Wolfenbarger et al., 2022). Frazil ice is composed of loose, randomly arranged ice crystals that form in supercooled turbulent water (Figure 3), and congelation ice forms on the bottom of an established ice cover. During ice formation in marine systems, brine is rejected at the ice-ocean interface, resulting in increasing transport of salt, chemicals, and organic components to depth that can impact subsurface circulation (e.g., Filchner-Ronne Ice Shelf in Antarctica; Hatterman et al., 2021). In addition, polynyas, open ocean areas in ice-covered seas, are regions of salt rejection into the underlying ocean as ice forms, a mechanism that can entrain chemical and molecular components at the ice-ocean interface (Greely et al., 1998; Arrigo et al., 2012; Meredith et al., 2019). Notably, Wolfenbarger et al. (2022) conclude that ice formed in the low temperature gradient environment beneath ice shelves in Antarctica may be a more relevant analog than sea ice for icy world shell dynamics, providing an alternate hypothesis for further Earth polar studies.
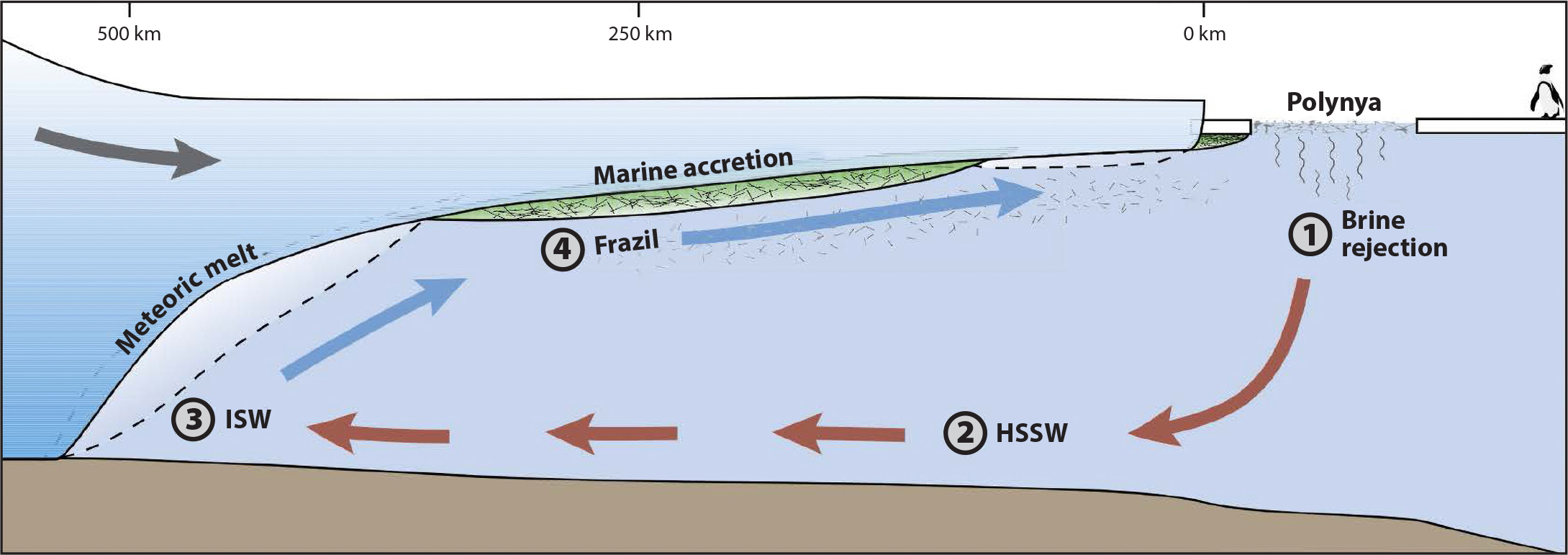
FIGURE 3. Earth’s polar regions with ice shelves and winter polynyas subject to climate change impacts have mechanistic analogs of processes potentially occurring on Europa. 1 = Brine rejection. 2 = HSSW (High Salinity Shelf Water). 3 = ISW (Ice Shelf Water). 4 = Frazil ice. Modified caption from Soderland et al. (2020) and modified image from Lawrence et al. (2018) . > High res figure
|
Subglacial lakes form in Antarctica and Greenland under kilometers of ice, with some interconnecting water flow between them (Wright and Siegert, 2012). Though these lakes have been isolated for tens of millions of years, scientists believe it is possible for organic life to exist in them. Microbes can live inside solid ice crystals deep in ice sheets and in underlying liquid water—the largest subglacial lake in Antarctica, Vostok, is known to harbor microbial life (for details, see Davies, 2020; German et al., 2022, and Glass et al., 2022, both in this issue). Subglacial lakes are ongoing areas of study for both Earth and planetary scientists. Also, recent studies in Subglacial Lake Whilians in Antarctica, located 800 m below glacial ice, indicate microbial communities within the water and underlying sediment zone. The authors conclude that relict marine-derived organic matter as well as mineral-derived energy from erosion of underlying rock material and subsequent release of reduced chemical species can support a diversity of microbial life, a potential analog for icy worlds with oceans, such as Europa (Gill-Olivas et al. 2021).
The thick, icy shells of some ocean worlds most likely undergo fracturing and faulting at their surfaces due to planetary tidal stress. The interior of the icy shell may be less influenced by external stresses and instead be more subject to warming as compared to their surface and subsurface interfaces (German et al., 2022, and references therein). Earth system scientists can contribute knowledge of various mechanisms acting on the icy shells of other worlds by identifying key climate-related physical forcing factors that act on Earth’s cryosphere and at ocean-rock interfaces. In a complementary manner, planetary scientists could provide Earth system scientists with a better understanding of key processes occurring within and below thick ice cover on Earth. Studies of deep convection processes that impact salty water underlying icy shell-covered planets could enhance understanding of both ice sheet dynamics and sea ice production in Earth’s polar regions. In addition, studies of planetary rock-water interface activities, such as proposed venting of minerals to the overlying water at depth on some icy worlds, could inform studies of microbes that live on reduced compounds and could provide additional insight into hydrothermal and deep mantle processes on Earth.
Looking at both temporal and spatial environmental impacts of climate change is important to this topic. Workshop participants recommended support for research activities that provide cross-fertilization opportunities among Earth and planetary science initiatives relevant to better understanding of ocean worlds, including planet Earth. A key question is how conditions at Earth’s ice-ocean interface might compare to those beneath the icy shells of ocean worlds, specifically related to pressure, temperature, and composition. Looking at Earth’s analogs may be key. Future work by the planetary and Earth-focused sea-ice communities should include understanding the marine and terrestrial processes that can be extrapolated to icy worlds, including modeling studies (Arrigo, 2022, and Glass et al., 2022, both in this issue). Because pressure governs temperature at the ice-ocean interface, the mechanisms at ice-ocean and ocean-rock interfaces have the highest potential for evaluating change at variable timescales on Earth and on other ocean worlds.
2. How can remote sensing and in situ measurements inform our understanding of planetary change, especially via polar systems and in the deep sea?
On planet Earth, autonomous remote sensing by satellites, in situ platforms, and other tools is necessary for studies of the under-ice environment, liquid water (oceans, deep lakes), and sediment/geological interfaces (coasts, ocean bottom, hydrothermal vent systems; Hendrix et al., 2019; Chirayath et al., 2022, in this issue). So equipped, Earth system scientists can provide past and present Earth measurements from polar and deep ocean systems that are relevant to planetary exploration in ocean worlds. Planetary science also requires autonomous capabilities for investigations into extreme environments (low and high temperature, ice cover/liquid subsurface systems, high pressure) for which technological developments would benefit current Earth system analyses (Chirayath et al., 2022, in this issue). Hand and German (2018) suggest development of a strong robotic program to directly investigate the surfaces and interiors of ocean worlds, including those of Earth. For planetary exploration, initial satellite and lander technology should be employed (Figure 4). Currently, efforts for two fly-by missions are ongoing: NASA’s Europa Clipper and the European Space Agency’s Jupiter Icy moons Explorer (JUICE) that will provide necessary data for deployment of future landers to look for evidence of life recorded on the surface of Europa (Howell and Pappalardo, 2020).
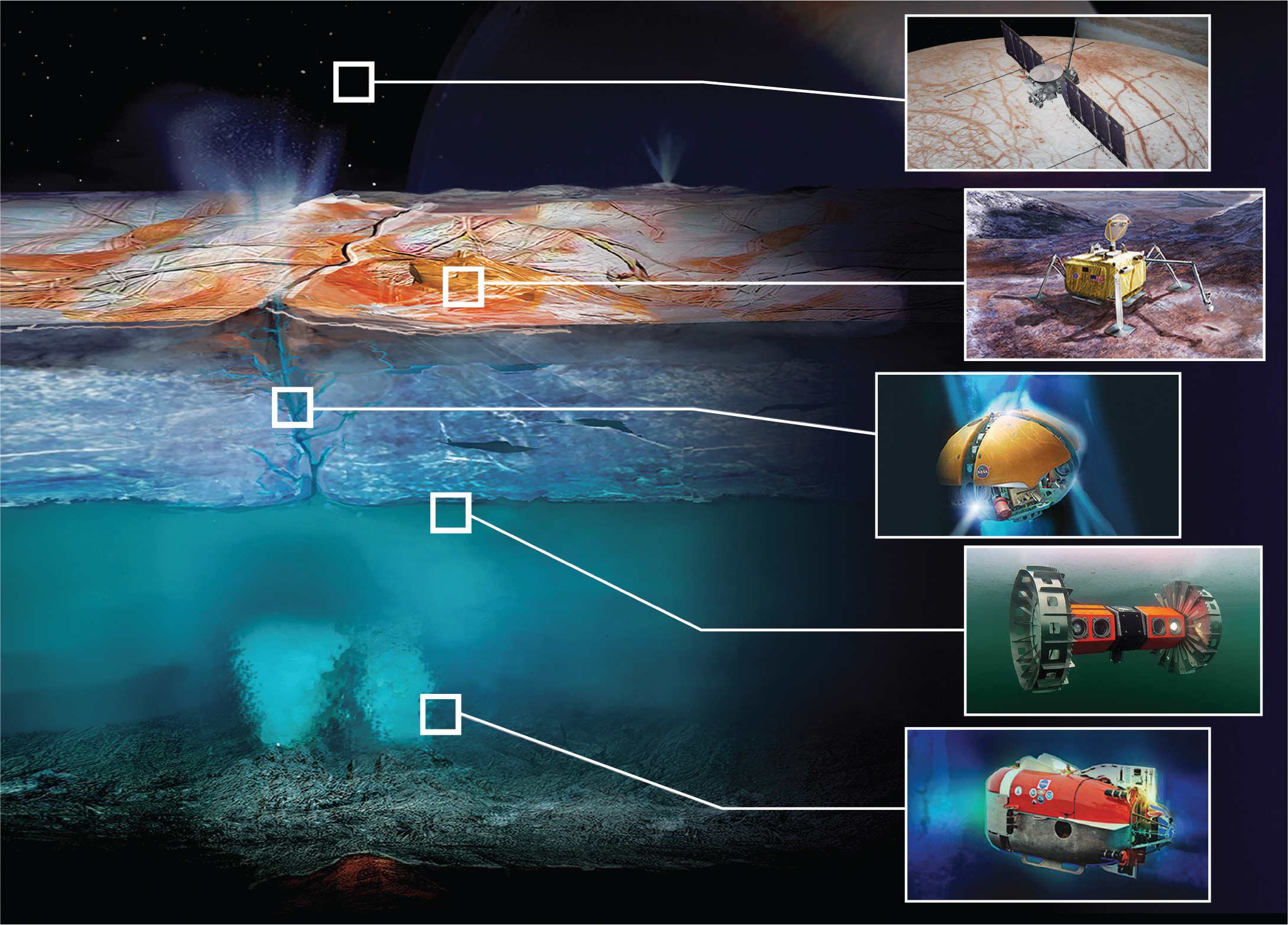
FIGURE 4. A schematic of required technological developments for future exploration of ocean worlds, inclusive of both oceanographic and planetary science collaborations. Initially, planetary ocean exploration would involve sending spacecraft and landers to icy worlds (top right two images). Subsequent investigations beneath the icy shells of ocean worlds will require testing of equipment in Earth’s ocean (lower right images), including continued development of melt probes to pass equipment through thick ice and deployment of autonomous underwater vehicles capable of collecting and analyzing scientific data and transmitting their results back to laboratories on Earth (see Chirayath et al., 2022, in this issue for details). Caption modified and figure from Hand and German (2018). > High res figure
|
The actual exploration phase of these distant oceans will require initial intensive testing of equipment in Earth’s ocean by including continued development of melt probes to pass equipment through thick ice (Chirayath et al., 2022, in this issue). Subsequent deployment of autonomous underwater vehicles capable of collecting and analyzing scientific data and transmitting their results back to laboratories on Earth is required (Figure 4; Hand and German; 2018, Hendrix et al., 2019). Technological development and testing on Earth will be the first phase of this exploratory robotics program. For example, ongoing Earth studies have used the autonomous vehicles to study the under-ice environment, the water column, and deep-sea hydrothermal vents, the latter under high pressure and low temperature conditions. However, there is a need for development of smaller and lighter autonomous vehicles to study Earth’s deep-sea systems as well as those of planetary icy worlds. For example, scientists at the Woods Hole Oceanographic Institution developed the lightweight (~250 kg), autonomous underwater vehicle Orpheus to navigate deep-sea systems. It is designed to identify features on Earth that could eventually be useful in identifying similar features on planetary icy world oceans and seafloors. The use of Terrain-relative navigation developed for exploration on Earth was instrumental in helping NASA’s Mars 2020 Perseverance rover touch down on the planet and map the Martian landscape autonomously. Testing of instruments used to further understanding of deep-sea hydrothermal vents will continue to expand our knowledge base of non-photosynthetic energy use for biological growth. In addition, studies that access subglacial lakes in polar regions will facilitate technological developments and our knowledge base for planetary expeditions coincident with a better understand of our own Earth system, including the impact of climate change on the cryosphere. Further details on technology are outlined in Chirayath et al. (2022) and Glass et al. (2022), both in this issue.
3. How does environmental change impact biological processes, and what are the feedbacks?
Human populations depend on biological processes for supplying oxygen to our environment and for producing food. Thus, environmental and food security are key components to human existence. The search for biological life beyond Earth and understanding humankind’s role in the overall planetary system is a basic human interest. An important goal for Earth and planetary scientists is to understand how environmental change impacts biological processes, from organic molecular development to single and multicellular growth (and destruction). Collaboration between these two disciplines is key to gaining this understanding.
Earth’s polar regions, which are most relevant to planetary studies, are rapidly changing as global temperatures rise. Ice production and melt at the ice-ocean interface influence large-scale ocean circulation patterns, which in turn affect the climate system (Meredith et al., 2019) as well as ocean biology and ecosystem structure (Olsen et al., 2017; Kauko et al., 2017). First-year sea ice that survives through the summer in the Arctic and grows thicker over the winter is then known as multiyear ice. Unlike seasonal ice, multiyear ice hosts fewer microbial sea ice communities and under ice phytoplankton (Kauko et al., 2017; Olsen et al., 2017), although habitable niches are observed at the ice-ocean interface in the Arctic and Antarctic (Bowman and Deming, 2010; Krembs et al., 2002, 2011; Campbell et al., 2021). Understanding the variability of life under limited light and varying biochemical conditions can inform the potential for biological life in low oxygen ice world oceans (e.g., Enceladus; Glein et al., 2015) as well as higher oxygen oceans (e.g., Europa; Hand et al., 2007; Russell et al., 2017). Thus, studies of the biology of Earth’s polar systems, including in subglacial lakes, can provide information about these types of habitats. Freshwater glaciers and ice sheets that are melting under increased global temperatures can release chemical components and organic material to the ocean that are then available for biological life systems. Of note was the discovery of sea anemones, multicellular filter-feeding organisms, ~65 km from the open ocean at the underside of the Ross Ice Shelf in Antarctica, which is ~260 m thick. Advected carbon and/or associated microbiomes are thought to provide the energy required by these organisms (Daly et al., 2013; Murray et al., 2016). Changes in ocean productivity due to environmental change could directly impact the level of carbon export to support life under the ice shelves.
In the deep sea, hydrothermal systems that vent at the seafloor are also key areas for cross-linked Earth and planetary studies relevant to deep ocean dynamics (German et al., 2022, in this issue). Hydrothermal vents on Earth release reduced chemicals at the rock-ocean interface that are used by chemosynthetic microbes to produce organic material. Chemotrophic bacteria convert hydrogen sulfide venting from rock into organic material that provides food for vent organisms such as tube worms. This process provides a potential analog to some subregions within oceans of icy worlds. The next section briefly outlines analogs on Earth of potential icy world systems and the potential impacts of climate change on these processes.
ANALOGS ON EARTH TO ICY WORLDS THAT ARE INFLUENCED BY CLIMATE CHANGE
Polar environments on Earth are key analogs of the physical and chemical conditions thought to exist in ice-covered oceans of planetary bodies (German et al., 2022, and Glass et al., 2022, both in this issue, and references therein). Terrestrial lakes can be found beneath thousands of meters of glacial ice in Antarctica and in ice-covered regions of Greenland in the Arctic (Siegert et al., 2016; Skidmore et al., 2019). Mechanisms for their formation are relevant to mechanisms that influence deep circulation patterns and associated processes that are subject to large-scale climate drivers (Carter et al., 2007). Studies of subglacial lakes under kilometers of ice in West Antarctica indicate that subsurface systems have variable geochemistry, oxygen levels, and biology (Murray et al., 2016; Davis et al., 2019; Skidmore et al., 2019). Similarly, the many subglacial lakes in Eastern Antarctica, also under kilometers of thick ice, are known to have variable geochemical levels, temperatures, and life forms, the latter not supported by photosynthesis (Siegert et al., 2016). Understanding mechanisms of chemical and life processes under Earth’s ice shelves are pertinent to understanding key processes influencing icy world oceans.
Studies of hypersaline lakes beneath the Devon Ice Cap (maximum ice thickness of 880 m) on eastern Devon Island, Nunavut, Canada, in the Arctic have evaluated key sub-ice processes, within both subsurface hypersaline lakes and brine plumes (Rutishauser et al., 2018). Using survey radar, gravity, and magnetics, the hypersaline subglacial lake complex was discovered within an evaporite-rich sediment sequence that likely acts as the solute source for the brine that may contain isolated microbial habitats. These saline subglacial lakes could thus act as analogs for potential ice-covered brine lakes and lenses on ice covered planetary bodies that are fueled by mantle release of chemical components, similar to hydrothermal vents on Earth.
In addition, joint Earth-planetary science studies of ice-ocean interactions and frazil ice development within polynyas, especially those that form in winter, may be useful for evaluating key processes at this important interface that are impacted by climate warming. Ito et al. (2019) found favorable conditions in winter during brine formation and salt rejection into the ocean that allowed the resuspension and freeze-up of sediment components within frazil ice. This mechanism could entrain chemical and molecular components at the ice-ocean interface or produce a subsurface pool of material in the upper ocean region in icy worlds that could act as potential sites for chemosynthetic life. Alternatively, Wolfenbarger et al. (2022) suggest that congelation ice produced in low temperature gradients may be more relevant to understanding icy world shell dynamics than sea ice studies. Ongoing climate change will impact ice composition and associated growth mechanisms on Earth; thus, studies of variable mechanisms for ice formation and salt extrusion should provide valuable information on icy world systems.
The availability of mineral-rich water in both the high Arctic and around Antarctica, at the ice-ocean interface, beneath floating ice shelves, or within deep-sea environments may influence the development of microbial life and multicellular organisms at these locations. Variable temperature and pressure regimes influence the growth of single-cell and multicellular forms of life under low or no light conditions (Campbell et al., 2021). Warming of seawater resulting from climate change is influencing processes at the ice-ocean interface in polar regions (Meredith et al., 2019; Perovich et al., 2020; Timmermans and Labe, 2020; Timmermans et al., 2020; Arrigo, 2022, in this issue). Study of ice-ocean processes can be valuable to joint Earth-planetary studies through both field studies and technological advancements.
Fluxes of elements through deep ocean sediments and at hydrothermal vents at mid-ocean ridges help support life (Hand et al., 2007; Van Dover and Task, 2000; Hoehler and Jørgensen, 2013; Gill-Olivas et al., 2021; German et al., 2022, in this issue). Many of the fundamental properties of ocean water (temperature, pressure, pH, reduction potential, salinity) and the availability of key nutrients (carbon, nitrogen, phosphorus, sulfur, iron) and energy in this analog environment may be modified by climate change. Colored dissolved organic matter (CDOM), humic substances, and clathrate deposits in polar regions are all important for sustaining microbial life (Glass et al., 2022, in this issue, and references therein) and are vulnerable to climate change as warming temperatures melt the ice matrix surrounding these substances.
Recent laboratory studies that applied destructive stress to extremophile bacteria at high temperatures resulted in the release of small fragments of carbohydrates, proteins, and lipids, potentially providing insight into the origin of organic matter on icy planets (Salter et al., 2020). This study undertook pyrolysis of organic material to simulate release of organic molecules within planetary plumes extruded from the icy shells of Saturn’s moon Enceladus and Jupiter’s moon Europa after collision with a spacecraft. A study at Lost Hammer spring on Axel Heibert Island in the Canadian Arctic suggests that lipids within Europa’s proposed mineral-rich subsurface ocean may be forced upward by pressure differences and penetrate the icy shell through fissures and could subsequently freeze on the moon’s surface and be detectable by satellite sensors (Cartier, 2022; Moreras-Marti et al., 2022). This Arctic site is a strong candidate for studies of microbial life within a cryovolcanic eruption, similar to hydrothermal vent processes in the deep sea on Earth and a potential process on Europa. Studies of key mechanisms at the ice-ocean and rock-ocean interfaces on Earth that influence the production and transport of carbon products in the salty ocean and through ice matrices in polar regions will increase understanding of potential processes occurring at ice-ocean interfaces on icy worlds.
Figure 2 summarizes the key interfaces and potential processes described for icy shell dynamics of Europa based on Earth studies. The rock-ocean interface could release key mantle chemical and mineral components (e.g., silicate) to produce a salty ocean above it, similar to Earth’s seafloor hydrothermal vents. The ice-ocean interface in the hard Europa icy shell could potentially hold a subsurface matrix of variable structure, similar to Earth’s cryosphere, which may be modified by climate change. Such an internal cryosphere matrix with variable stress levels and density could allow both upward motion for surface venting of ocean plumes and/or downward exchange of concentrated components to the ocean below. Depending on the salt content, the drained icy shell water could contain chemical and organic components previously released from depth that can be used to support life. Hydrothermal vents at the deep rock-ocean interface, as well as subsurface lakes below the ice-ocean interface, provide viable Earth analogs that could support life. Notably, a mechanism similar to winter polynya formation in polar regions on Earth, where salt is extruded as ice forms, can act as a concentrating mechanism for chemical and organic materials; a similar mechanism could be feasible in other icy worlds. These processes, subject to impacts from climate change on Earth, could help identify key mechanisms at work on icy worlds. Conversely, the study of icy worlds can inform current and past processes on Earth.
CONCLUSION
To accelerate research related to studies of icy ocean worlds requires developing synergistic research questions and collaborations between planetary scientists and Earth’s terrestrial and ocean science communities. Bodies with icy shells could harbor subsurface oceans and potentially biological components indicative of life. Earth’s polar regions and deep-sea hydrothermal vents can be used as analogs for studying processes potentially occurring in other icy worlds and can provide a significant focus for collaboration between Earth and planetary scientists. Studies of ongoing changes resulting from global warming on Earth, such as those at the ice-ocean interface, within subsurface glacial lakes, and in ice sheet dynamics can all provide the information necessary to evaluate the physical dynamics of other ice-covered worlds. Studies of deep-sea hydrothermal vents, chemical exchanges at the rock-ocean interface, and chemosynthetic processes influencing microbial and multicellular life are relevant to processes in other worlds that potentially harbor subsurface oceans below their icy shells. Studies of terrestrial and marine analogs in polar regions and in the deep sea on Earth can provide a better understanding of potentially life-sustaining process in other stellar bodies, such as Europa. The development of advanced satellite sensors, landers, and robotics for studies on other world will benefit Earth research as well.
Similarly, planetary studies of both rock-ocean and ice-ocean interfaces in icy worlds can help Earth scientists better understand key processes on Earth, in particular, in environments that have extreme temperatures, pressures, and variable salinity realms. Complementing the physical sciences studies, planetary investigation of key minerals important for sustaining life on Earth, and the associated energy transformations, can provide valuable insight to Earth scientists who study cryospheric and deep-sea processes. Mechanisms that influence any component of the carbon cycle on icy worlds and associated microbial life are important topics for Earth studies, especially under projected climate change scenarios that could have a major impact on the status and change in Earth’s ecosystem.
Finally, a joint working group consisting of scientists from both Earth system and planetary communities could discuss the status of and gaps in understanding related to key processes pertinent to sustaining life on Earth and in other parts of the solar system. The joint working group could also identify opportunities for testable ideas based on the Earth system that would benefit planetary studies and further the development of autonomous robotic equipment. This joint effort would allow development of a forward-looking strategy to advance our knowledge of ocean worlds with icy shells in our solar system.
Acknowledgments
This paper was based on discussions initiated at the December 2019 NASA Ocean Science Across the Solar System workshop, co-chaired by Laura Lorenzoni and Mary Voytek. Input during the meeting was provided by Samuel Howell (Jet Propulsion Laboratory) and Alejandro Soto (Southwest Research Institute), along with other participants in the workshop as reported in other papers of this special issue. Ashley Kleinman kindly assisted in preparing the manuscript for publication. Financial support for participating in the 2019 workshop was provided by NASA and support for developing this paper was provided by NSF OPP grant 1917469. Further Ocean World Resources are available at: https://solarsystem.nasa.gov/news/1440/ocean-worlds-resources/.