Introduction
This paper is intended primarily as an update to the previous assessment of Arctic sea ice published a decade ago in Oceanography (Perovich et al., 2011). Over that decade, substantial changes in Arctic sea ice have been observed (e.g., Meier et al., 2014; Barber et al., 2017), with declining sea ice cover being one of the clearest indicators of change, along with thinning of the ice cover (Kwok, 2018). Spring melt is occurring earlier and freeze-up is trending later, allowing the ice-ocean system to absorb more solar radiation and increasing the energy input into the Arctic. At this point, it is highly likely that ice-free conditions will emerge in September by the middle of the century (e.g., Notz and SIMIP, 2020). It is only under limited future emissions scenarios that the likelihood of largely sea ice-free conditions during summer can be avoided on a regular basis. The impacts of sea ice loss are myriad within the Arctic: warmer ocean waters, longer fetch, more frequent storms, and increased coastal erosion, along with associated effects on the Arctic ecosystem and human activities in the region. The loss of sea ice also amplifies Arctic warming, impacting Greenland ice mass loss and permafrost thawing. The ramifications of sea ice loss outside the Arctic are uncertain, with conflicting evidence of connections to more extreme weather events in the mid-latitudes.
Observing
Ice Concentration and Sea Ice Extent
A series of satellite-borne passive microwave sensors provides a consistent and nearly complete long-term record of sea ice concentration and extent since November 1978. Sea ice extent (sum of the area with at least 15% concentration) has been a workhorse in assessing the state of the ice cover because of the available long, consistent record. Several time series of extent have been produced from passive microwave brightness temperatures via various empirically derived sea ice concentration algorithms (e.g., Comiso, 1986; Spreen et al., 2008; Lavergne et al., 2019). Here, we use the extent record from the US National Snow and Ice Data Center (NSIDC) Sea Ice Index (Fetterer et al., 2017) derived from NASA Team algorithm concentration fields (Cavalieri et al., 1999); extent here is defined as the total area where concentration is greater than 15%. The concentration product begins in November 1978 (Cavalieri et al., 1996), with the most recent data (for 2021 in this manuscript) augmented by near-real-time processing (Maslanik and Stroeve, 1999).
Sea ice concentration and extent are declining everywhere in the Arctic, with the most pronounced losses in summer occurring within the Beaufort, Chukchi, East Siberian, and Laptev Seas, and the largest ice losses in winter within the Barents Sea and the Sea of Okhotsk. (Figure 1a,b). Much of the concentration trend is due to complete loss of ice (i.e., decline in extent and retreat of the ice edge), but some areas within the ice pack are also trending toward lower concentration. This suggests a less compact ice pack that allows more solar absorption during summer and less resistance to wind and other dynamic forcing.
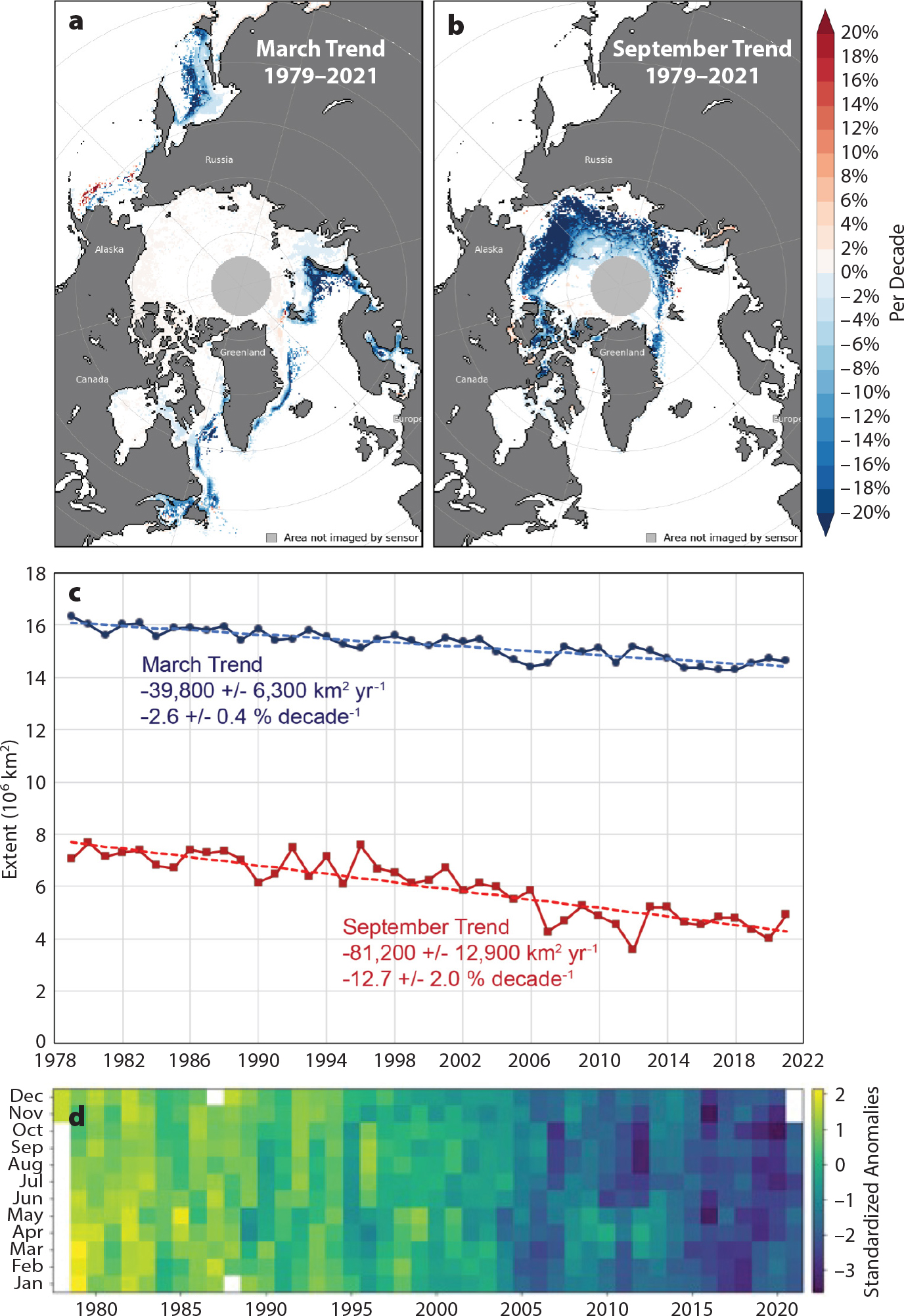
FIGURE 1. Arctic sea ice trends for 1979 to 2021. Percent per decade (relative to the 1981–2010 average) concentration trend for (a) March and (b) September. (c) Percent per decade (also relative to the 1981–2010 average) extent trends for March and September with linear trend lines. (d) Standardized anomalies in Arctic sea ice extent relative to the 1981–2010 long-term average. > High res figure
|
The sea ice extent trend in September, when the annual minimum occurs, is –12.7% per decade, while winter trends are smaller but still statistically significant (p <0.05) (Figure 1c). Trends for 1979–2021 are negative and statistically significant for all months, with extents since 2005 consistently well below normal, particularly during spring and autumn (Figure 1d). The largest departures from average conditions recently have occurred in October, with the largest negative anomaly being the October 2020 extent that was 3.7 standard deviations below the 1981–2010 mean.
Despite statistically significant negative trends, the overall linear trend is marked by strong interannual and decadal variability. Nevertheless, each decade’s sea ice extent has been lower than that of the previous decade. The most recent decade has seen particularly extreme September extents with the record low extent reached in September 2012 (3.39 × 106 km2), and the second lowest extent occurring in September 2020. Overall, the last 15 years (2007–2021) have the 15 lowest September extents in the 43-year (1979–2021) satellite record. However, the trend has been relatively flat over those years (–8,200 ± 57,400 km2 yr–1).
Looking at sea ice extent decade by decade, the variability is evident, with the strongest trend during the 2001–2010 decade and the weakest trend in the past decade, 2011–2020 (Table 1). However, most of the decadal trends are not statistically significant due to the short time period of the data. But change in extent is evidenced by the 2011–2020 decade being nearly 1 × 106 km2 lower than the previous decade and almost 2.5 × 106 km2 below the first complete decade in the record (1981–1990).
TABLE 1. Statistics on September sea ice extent. Trends are given with two standard deviation ranges; significant trends (p <0.05) are in bold. Percent trends are relative to a 30-year (1981–2010) climatological average. > High res table
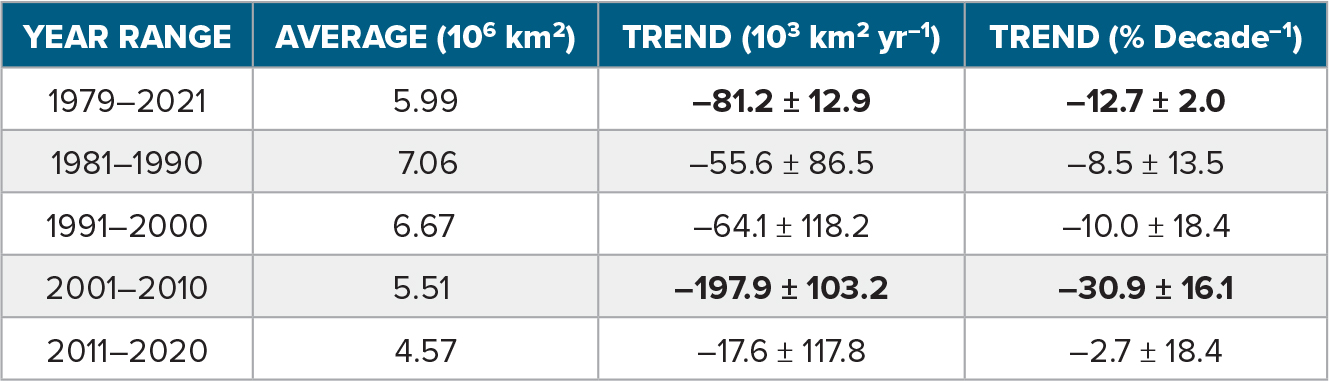
|
Ice Age
Sea ice age provides yet another long-term indicator of change in the Arctic. Age is tracked via Lagrangian parcels (Tschudi et al., 2020), and a data product (Tschudi et al., 2019a,b) for age is available beginning in 1985. Older, level ice is generally thicker than younger ice (ignoring dynamic thickening), so age provides a general proxy for thickness. Changes in the age distribution within the Arctic indicate a substantial loss of older ice. While multiyear ice (ice that has survived at least one summer melt season) and >4-year-old ice extent have declined almost since the beginning of the record, the last 10 years have seen an almost complete disappearance of ice >4 years old, with extents persistently below 500,000 km2 since 2012 (Figure 2). The total area of multiyear ice has shown interannual variability since the record low extent in 2012, but it has continuously been well below values seen before 2007. Simply put, sea ice is not remaining in the Arctic as long as it once did.
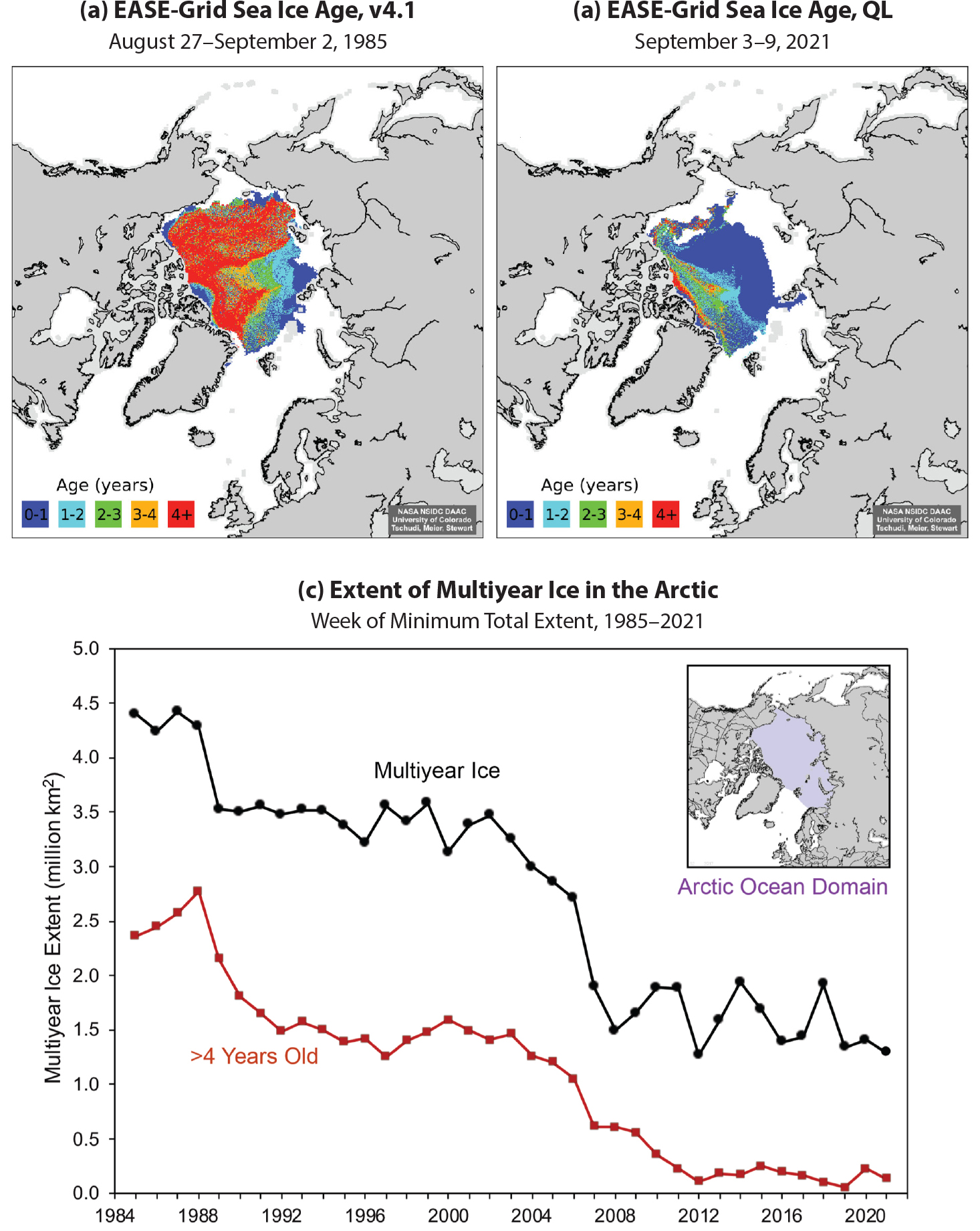
FIGURE 2. Weekly average sea ice age field from the end of summer (week before the annual minimum total extent) for (a) 1985 (from Tschudi et al., 2019a) and (b) 2021 (“QL” = QuickLook version from Tschudi et al., 2019b). (c) Extent of age of multiyear ice (black) and >4 year old ice (red) within the Arctic Ocean domain (inset) for 1985 to 2021. Figure from Meier et al. (2021). > High res figure
|
There are two apparent reasons for this shorter lifetime of ice in the Arctic. One reason is faster ice motion (Kwok et al., 2013). This increase in speed is not explained by increasing wind forcing or currents, but rather it is a greater response to forcing by the younger and thinner ice cover, as well as a less compact ice pack, as noted above in the concentration trend data. This leads to increased area export (Smedsrud et al., 2017), though volume export appears to decrease due to thinning (Spreen et al., 2020). In some respects, this can be thought of as a potential positive feedback mechanism: thinner and less compact ice (due to warming) responds more to forcing and moves faster, exiting the Arctic sooner, which results in a thinner ice cover.
The other aspect leading to less older ice is in situ melting. In particular, in the Beaufort and Chukchi Seas, where ice once circulated clockwise in the Beaufort Gyre, the ice age data show that much of the ice is melting out during summer in that region. This may be due to a combination of warmer ocean waters and a less compact ice pack (which may in turn be due to a thinner, more dynamic ice cover).
Ice Thickness and Snow Depth
While we now have over 43 years of consistent observations of sea ice area and extent, we do not have a similarly long-term data record of sea ice thickness. Thickness, when combined with ice extent or area, provides estimates of ice volume, arguably a more important metric of the overall amount of ice being lost in the Arctic Ocean. Our earliest observations of sea ice thickness were primarily based on submarine upward-looking sonar data collected in the 1980s and 1990s (NSIDC, 1998). In regard to satellite-based approaches, most are based on using radar or laser altimeters. Neither of these technologies actually measure the sea ice thickness, but instead they measure either the radar freeboard, or in the case of laser altimeter, the snow + ice freeboard relative to the water surface. Together with estimates of snow depth, and snow, ice, and water densities, sea ice thickness can then be inferred by assuming the sea ice and its overlying snow cover are in hydrostatic equilibrium (e.g., Laxon et al., 2013). In the case of radar altimetry, a further assumption as to the location of the dominant backscattering surface is needed. This is often assumed to be the snow/ice interface at Ku-band, though this assumption is likely only valid for a cold snow pack over multiyear ice. Altimetric records have higher uncertainties for thinner ice. For thin ice, the use of passive microwave brightness temperatures at L-band have also been used (e.g., Kaleschke et al., 2012), but these estimates are limited to a thickness of about 50 cm, though they can be combined with Ku-band radar altimeter data from ESA’s CryoSat-2 mission for an optimal estimate (Ricker et al., 2017).
The first estimates of sea ice thickness for a substantial part of the Arctic (up to 81.5° N) came from the ERS-1 radar altimeter satellite for 1993 to 2001 (Laxon et al., 2003). This was followed by NASA’s Ice, Cloud, and land Elevation Satellite (ICESat) laser altimeter mission; however, because of technical problems with the lasers, ICESat only provided snapshots of Arctic sea ice thickness during spring and autumn from 2003 to 2009. Since 2010, CryoSat-2 has provided nearly pan-Arctic observations of ice thickness. Beginning in 2018, NASA’s ICESat-2 laser altimeter began providing complementary estimates to CryoSat-2. While these different satellite missions offer glimpses into sea ice thickness variability and change, it remains challenging to blend these data into a consistent record of ice thickness. This in part stems from different sensors (i.e., laser vs. radar altimeter), spatial resolution (i.e., larger footprint of ERS-1 vs. CryoSat-2 generates inconsistencies in the dominant scattering surface observed), differences in assumptions about snow/ice densities, and differences in snow depth estimates used in thickness retrievals. Because snow depth has not yet been accurately observed by satellite, a climatology for snow depth is often applied. Yet, using a climatology can lead to large biases in sea ice thickness trends, especially in the marginal ice zone. There, snow depth is observed to be declining, in part due to later autumn freeze-up and thus less time for the snow to accumulate on the ice (e.g., Stroeve et al., 2020). Figure 3 shows an example of trends in April ice thickness from 2011 to 2020 from the CryoSat-2 data record. In this example, ice thickness retrievals using snow depth and density from Liston et al. (2020) were compared against those using a snow depth and density climatology (e.g., Warren et al., 1999). Conversion of radar freeboard to thickness was based on an algorithm from Landy et al. (2020).
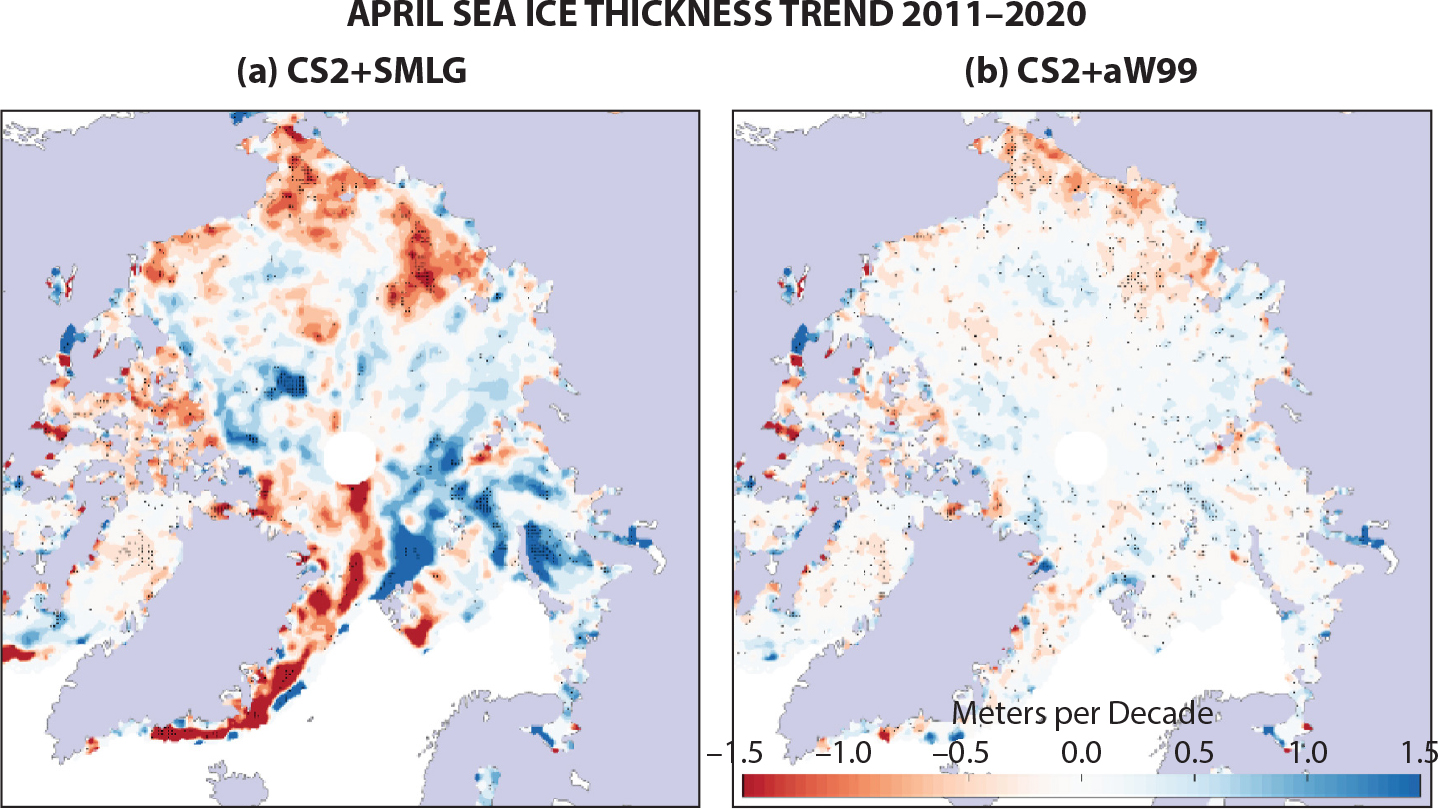
FIGURE 3. Trends in April sea ice thickness in meters per decade between 2011 and 2020 derived from CryoSat-2 (CS2) freeboard retrievals using the Landy et al. (2020) algorithm with (a) SnowModel-LG (SMLG) snow depth and density (Liston et al., 2020) and (b) snow depth and density climatology (adjusted W99; Warren et al., 1999; Laxon et al., 2003). Stippling indicates significance at p <0.05. Figure provided by J. Landy (University of Bristol). > High res figure
|
What is clear is that thickness trends are overall larger in magnitude when using a dynamic snow loading data set versus a fixed climatology, and there are some spatial pattern differences in regions with positive or negative thickness trends. However, many regions where the trends are statistically significant at the 95% confidence interval are broadly similar regardless of which snow data set is used. From this we can conclude that during the CryoSat-2 period, end of winter ice thickness is declining most strongly in the Beaufort, Chukchi, East Siberian, Laptev, Lincoln, and East Greenland Seas as well as within the Canadian Arctic Archipelago and Baffin Bay, but thickness is increasing north of the Canadian Arctic Archipelago and in the Barents and Kara Seas (Figure 3).
For a longer-term perspective, Mallett et al. (2021) showed that using a newly developed dynamic snow depth and density product (Liston et al., 2020), ice thickness declined 60%–100% faster between 2002 and 2018 compared to using the Warren et al. (1999) snow depth and density climatology. The most recent synthesis of thickness changes using earlier submarine and mooring data together with measurements from electromagnetic induction sensors on helicopters and aircraft, and airborne and satellite lidar data (Lindsay and Schweiger, 2013) found that between 1975 and 2012, the mean ice thickness declined from 3.59 m to 1.25 m. These ice thickness changes are consistent with the shift from an Arctic Ocean dominated by multiyear ice to one dominated by first-year ice.
As noted above, knowledge of snow depth is essential to retrieve ice thickness from altimetry. Thus, it is useful to briefly comment on progress in monitoring snow depth. The first satellite estimates were based on use of passive microwave brightness temperatures to retrieve snow depth over first-year ice (e.g., Markus et al., 2011). This was later extended to also include multiyear ice (e.g., Rostosky et al., 2018). Another satellite-derived method is based on the assumption that radar backscatter at Ka-band comes from the snow surface, while that from Ku-band comes from the ice surface, and thus the difference between the two provides an estimate of snow depth (e.g., Guerreiro et al., 2016; Lawrence et al., 2018). This has been extended to using a combination of ICESat-2 and CryoSat-2 freeboards (e.g., Kwok et al., 2020). Other approaches attempt to model snow accumulation using atmospheric reanalyses combined with various levels of snow modeling sophistication (e.g., Blanchard-Wrigglesworth et al., 2018; Petty et al., 2018; Liston et al., 2020) in either a Lagrangian or Eulerian framework. The Liston et al. (2020) approach is currently the most sophisticated snow modeling system available for providing physically constrained estimates of snow depth and density. The different approaches provide differing magnitudes in total snow depth and trends, as well as spatial patterns (Zhou et al., 2021). However, most of the reanalysis-based approaches show negative trends in snow accumulation in the marginal ice zone (Figure 4), consistent with later ice formation (see next section). Slight positive trends in snow accumulation are seen north of Greenland and the Canadian Arctic Archipelago, with some data products stretching across the pole (see Zhou et al., 2021).
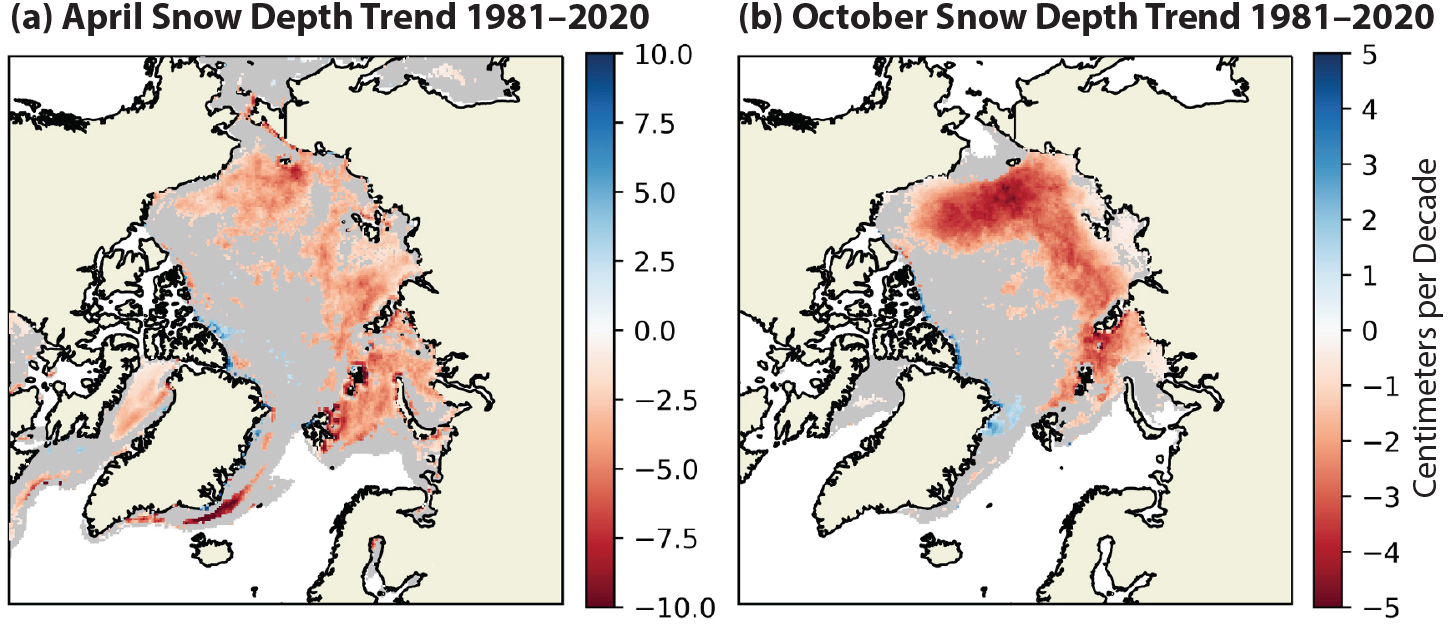
FIGURE 4. Snow depth trends for 1981 to 2020 during (a) spring (April) and (b) autumn (October). Only statistically significant trends (at p <0.05) are shown in color; gray indicates trends that are not significant. Figure provided by R. Mallett (University College London). > High res figure
|
Melt Onset and Freeze-up
The sensitivity of microwave emissivity to the presence of liquid water in the snowpack has also allowed for the mapping of changes in the timing of melt onset and freeze-up (e.g., Markus et al., 2009; Bliss and Anderson, 2018; Peng et al., 2018). As expected in a warming Arctic, the melt season is starting earlier than it once did, with the largest changes observed in the marginal seas of the Arctic, with trends on the order of 10–20 days earlier each decade (Figure 5). Slight delays in melt onset occur in the central Arctic (two to five days later each decade). Earlier melt onset has been linked to advection of warm, moist air masses into the Arctic (Kapsch et al., 2013; Mortin et al., 2016).
Trends in autumn freeze-up are in general larger than those of melt onset, with particularly large delays in freeze-up observed in the Beaufort, Chukchi, and East Siberian Seas (up to a month later each decade in the northern Chukchi Sea; Figure 5). Freeze-up is both a measure of when the surface refreezes and also when new ice forms. Despite more modest trends in melt onset compared to freeze-up, earlier melt onset lowers the surface albedo earlier in the melt season, helping to enhance the ice-albedo feedback (e.g., Stroeve et al., 2014). Earlier formation of melt ponds and open water areas results in absorption of more of the sun’s energy, in turn fostering more ice melt. The heat gained in the ocean mixed layer as a result of earlier melt onset and earlier ice retreat is strongly linked to the timing of ice formation and thus freeze-up (e.g., Stroeve et al., 2016, 2014).
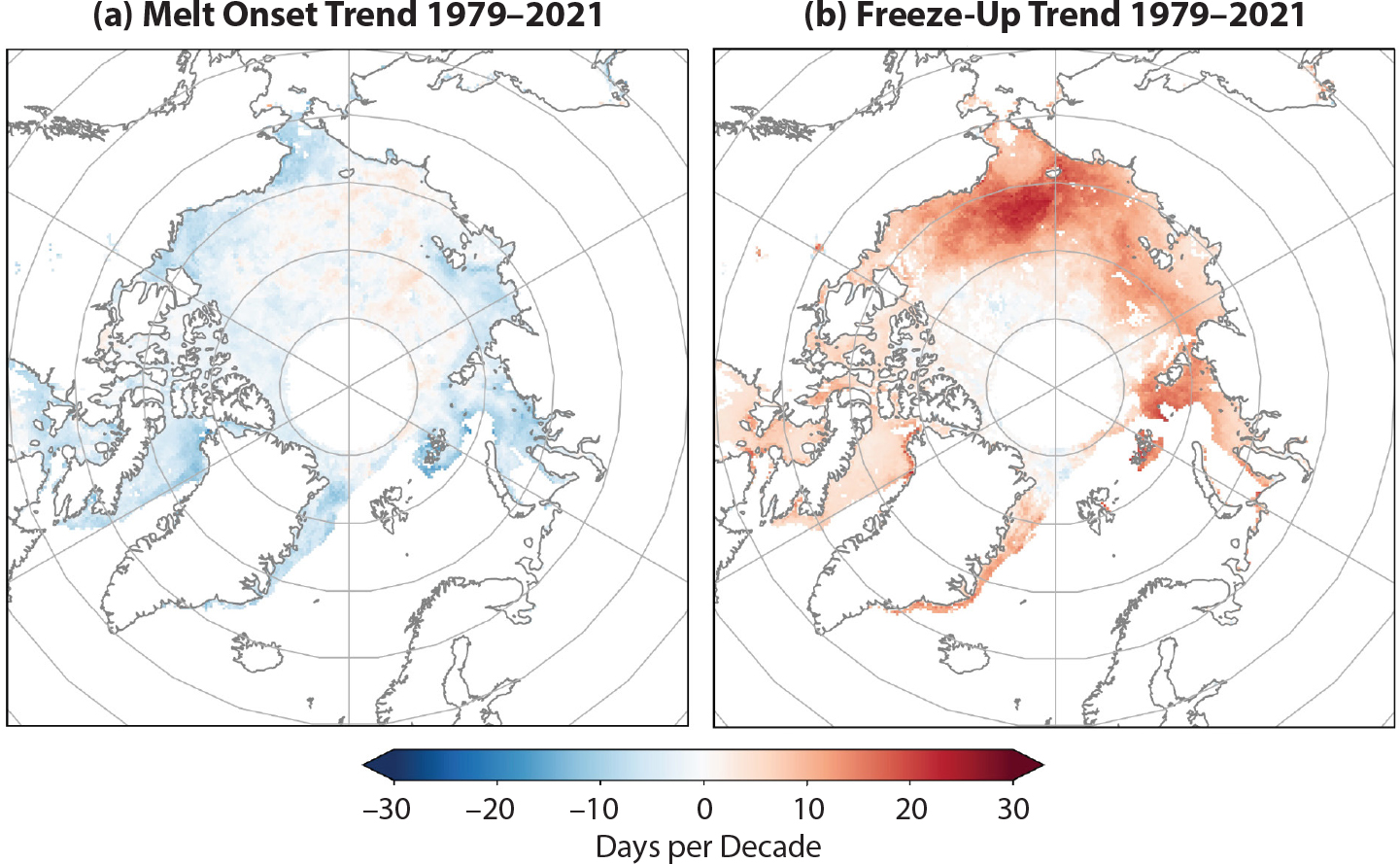
FIGURE 5. Melt onset (a) and freeze-up (b) trends. Data updated from Markus et al. (2009). > High res figure
|
Finally, earlier melt onset allows for earlier formation of melt ponds, and thus trends toward earlier melt pond formation would be expected. This may be especially important given the role melt ponds may play in the amount of ice left at the end of summer (e.g., Liu et al., 2015). Tracking of melt ponds with satellite data remains challenging given the relatively coarse spatial resolution of satellite data. However, in the past decade substantial progress has been made using optical satellite imagery, such as from NASA’s Moderate Resolution Imaging Spectroradiometer (MODIS) instrument (e.g., Tschudi et al., 2008; Rösel et al., 2012; Lee et al., 2020), as well as data from the Medium Resolution Imaging Spectrometer (MERIS) satellite (Zege et al., 2015). Data from each has produced melt pond estimates at different spatial and temporal resolutions, making an intercomparison between products difficult. For long-term trends, only Lee et al. (2020) have developed products through 2020, whereas the other products end in 2011 or 2012. Overall, no statistically significant trends toward earlier melt pond development are observed in any of the data products between 2000 and 2011, though Lee et al. (2020) show positive trends during July and August when the record is extended to 2020.
Drivers of Sea Ice Changes
While the overall long-term decline in Arctic sea ice extent is clear (Figure 1), how well a particular year tracks with the linear trend depends strongly on atmospheric circulation patterns (e.g., Parkinson and Comiso, 2013; Ding et al., 2019). Earlier studies show linkages between atmospheric modes of variability, such as the Arctic Oscillation (e.g., Rigor et al., 2002), and summer sea ice extent. However, in recent years, low summer extents have continued regardless of the atmospheric mode. One reason for this is that today’s Arctic ice is considerably thinner than it was four decades ago. Higher temperatures and a thinner ice cover serve to precondition the ice cover to be more sensitive to seasonal weather patterns (e.g., Babb et al., 2015). Thus, an unusually warm summer (e.g., Stroeve et al., 2008), or a strong cyclone (Parkinson and Comiso, 2013), can result in large reductions in both volume and extent regardless of the atmospheric mode. Conversely, a colder than average summer may reduce ice melt and permit a relatively thin ice cover to survive.
Another factor in sea ice change is warming of the ocean, which also acts as a positive sea ice-albedo feedback: loss of ice results in more solar absorption in the ocean and warming of the water, which melts more ice (e.g., Perovich et al., 2007). One study found a fivefold increase in summer solar heat absorption in the northern Chukchi Sea between 1987 and 2017 (Timmermans et al., 2018). There is also evidence in the Eurasian Basin that the halocline between the colder, fresher surface waters and the warmer, saltier Atlantic Water below is weakening and contributing to sea ice loss in the region (e.g., Polyakov et al., 2017, 2020; Ricker et al., 2021). Earlier snow melt onset and melt pond formation are also part of a positive feedback mechanism, as they decrease surface albedo and increase solar absorption by the ice (e.g., Perovich et al., 2007).
The variability in forcing and the changing Arctic sea ice response to that forcing make seasonal forecasting challenging. Forecasts of September sea ice with one- to three-month lead times have shown varying but limited skill (e.g., Blanchard-Wrigglesworth et al., 2015; Hamilton and Stroeve, 2016). Forecasting may be becoming more difficult with the thinner ice cover. When the Arctic Ocean was covered by thick ice, an unusually warm summer may have melted a relatively large volume of ice, but this would not have been reflected in a change in extent to the degree that it would be now.
There is still much to learn about the complex processes of the sea ice cover and their interactions with the ocean and atmosphere. While satellite data have greatly expanded our knowledge of these processes, field observations are still essential to validate satellite data and models and to better understand small-scale processes. One of the most momentous undertakings in the history of Arctic science occurred in the past decade: the Multidisciplinary Drifting Observatory for the Study of Arctic Climate (MOSAiC; Shupe et al., 2020). The German icebreaker Polarstern was frozen into the ice and drifted across the Arctic from October 2019 to September 2020, collecting ice, ocean, atmosphere, and biogeochemistry data through a full annual cycle. The data are still being processed and substantial results have yet to be reported. But the data collected promise to be a treasure trove for future understanding of the changing Arctic sea ice.
Though details of sea ice processes and interactions with the ocean and atmosphere are still not completely understood, the shrinking and thinning of Arctic sea ice has a clear fingerprint from rising concentrations of atmospheric greenhouse gases. Notz and Stroeve (2016) examined the linear relationship between September sea ice decline and cumulative CO2 concentrations. When this evaluation was expanded to all months of the year, it indicated that all calendar months demonstrate a clear linear relationship, though the relationship is strongest in September. Updating this analysis through 2021 shows that the linear relationship still holds today (Figure 6). Thus, the long-term fate of sea ice will be determined by which emission scenario (denoted in the IPCC AR6 Report as Shared Socioeconomic Pathways [SSPs]) is realized within Earth’s climate in the coming decades. Although the target for limiting global warming is 1.5°C, the warming in the Arctic will greatly exceed this amount, with warming as large as 6°C in autumn and winter. If the planet warms to 2.0°C, the warming will exceed 8°C in the Arctic (Figure 6b).
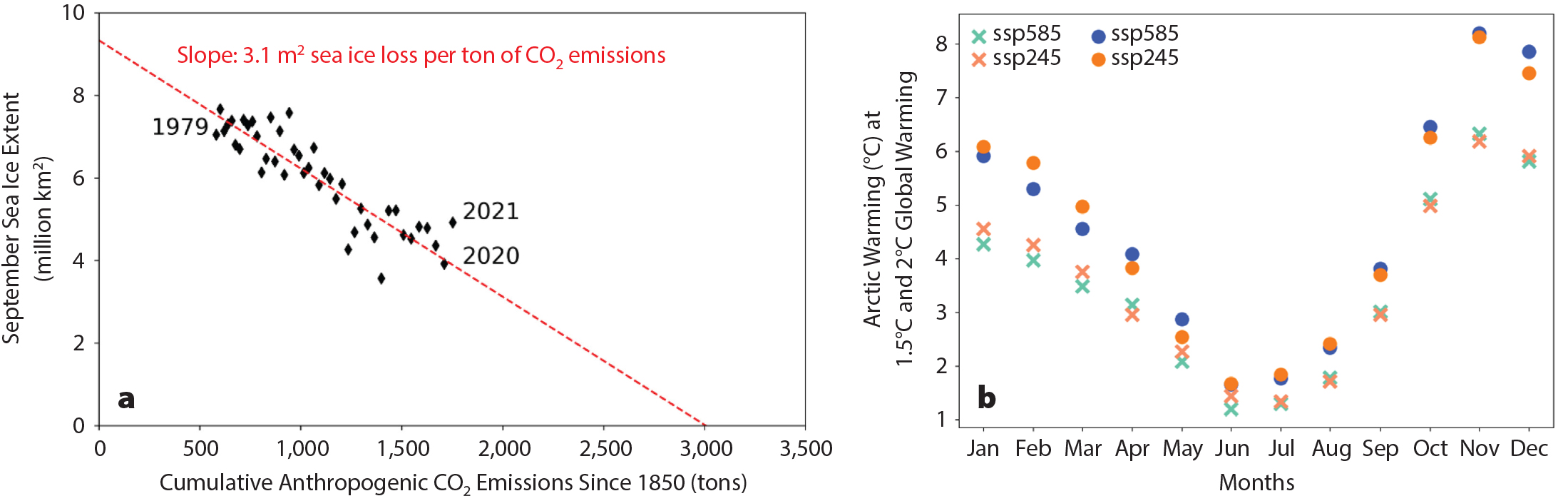
FIGURE 6. (a) September sea ice extent versus cumulative CO2 emissions for 1979 to 2021 and linear slope. (b) Monthly mean Arctic warming under two future emission scenarios from the latest round of climate model simulations, denoted in the IPCC AR6 Report as Shared Socioeconomic Pathway (SSP) 245 (orange) and SSP 585 (blue) under an annual global warming of 1.5°C (x’s) and 2.0°C (circles). Figure 6a updated from Notz and Stroeve (2016); Figure 6b provided by M. McCrystal (University of Manitoba). > High res figure
|
Impacts of Changes
The loss of sea ice has myriad impacts within the Arctic. A comprehensive assessment of such impacts is beyond the scope of this paper, but they are detailed in various assessment reports (e.g., AMAP, 2017) and other studies (e.g., Post et al., 2019). Here, we provide brief examples of some of the impacts.
Less sea ice has led to longer fetch, more coastal wave action, and, coupled with permafrost thaw, more coastal erosion (e.g., Overeem et al., 2011; Fritz et al., 2017), results that threaten Indigenous communities and other human infrastructure in the north. Earlier retreat and later advance of ice is opening up shipping routes, and as sea ice declines further, shipping through the Arctic will become more viable in the future (Mudryk et al., 2021).
The loss of ice has fostered earlier and more widespread phytoplankton blooms (e.g., Hill et al., 2018). Double blooms (Ardyna et al., 2014), as well as large under-ice blooms (Arrigo et al., 2012), have been observed in recent years. A lack of ice during Bering Sea winters resulted in substantial effects on the regional ecosystem, including seabird die-offs (e.g., Duffy-Anderson et al., 2019). There are also well-known negative impacts on the megafauna of the Arctic, such as polar bears (Pagano and Williams, 2021), although habitats are expanding for non-ice species, such as killer whales and some fishes (Stafford et al., 2022, in this issue).
While the impacts within the Arctic are clearly visible, the influence of sea ice and Arctic change outside of the Arctic is far more uncertain. Francis and Vavrus (2012) first proposed a connection between Arctic sea ice loss and warming and mid-latitude weather extremes via a slowing jet stream. Their analysis indicated a detectable change in the jet stream pattern that they related to the warming and sea ice loss. However, almost immediately, other studies found contradictory results (e.g., Barnes, 2013). Since then, myriad studies have provided contradictory information. Synthesis studies have tried to reconcile the conflicting research (e.g., Overland et al., 2016), but the debate continues, with studies both supporting (e.g., Cohen et al., 2021) and contradicting (Blackport and Screen, 2021) the hypothesis.
Summary
It is difficult to produce an assessment of Arctic sea ice because changes are happening so rapidly—this document will likely be out of date shortly after publication. In some ways, the story is the same as in the previous report published in Oceanography (Perovich, 2011): the rapidly changing Arctic is marked by sea ice loss. On the other hand, substantial developments have emerged in the past 10 years. There was a new record low September ice extent in 2012 and several other extreme low years since then. The oldest ice, already in steep decline 10 years ago, has virtually disappeared and shows no signs of recovery. Since 2011, there have also been substantial new observing capabilities, particularly from altimeters, providing the most complete satellite estimates of freeboard and thickness ever, though there remains important uncertainty in the retrievals (particularly due to snow properties). The Arctic sea ice is showing a consistent response to warming across the myriad observations: decreases in concentration and extent, a younger and thinner ice cover, earlier melt, and later freeze-up.
New projections of sea ice cover confirm an ultimate dependence on future emissions scenarios, though considerable uncertainty will continue in year-to-year variability. Extending the still relatively short records of observations of ice thickness and snow depth and reducing uncertainties in their estimates will help constrain model projections. And future improvements in models (e.g., parameterizations, vertical/horizontal resolution) should also yield more precise projections. A controversial line of research has emerged in the last 10 years, positing a connection between Arctic warming and sea ice loss and mid-latitude weather extremes. Despite numerous studies, the connection remains uncertain and debated within the scientific community. More data, particularly on weather extremes, and improved modeling may help to resolve this question in the future.
What is certain is the impact of sea ice loss within the Arctic. Even 10 years ago, the impacts of sea ice loss on the regional climate, local communities, and the ecosystem were clear and have become only more so since then.