Introduction
The coastal margins of Kalaallit Nunaat (Greenland) are unique regions shaped by the confluence of Atlantic and Arctic Ocean waters; the discharge of icebergs, meltwater, and sediments from the ice sheet; the formation, melt, and transit of sea ice; and the battering of extreme storms and katabatic winds. They comprise shallow continental shelves incised by deep troughs scoured by ice streams during glacial periods, and hundreds of glacial fjords, some over a kilometer deep, tens of kilometers long, and only a few kilometers wide. The interaction of ocean currents, glaciers, and winds gives rise to nutrient-rich waters and other conditions that support rich marine ecosystems, including a high density of seabirds, marine mammals, and fish (Figure 1). These coastal ecosystems, in turn, support people and communities whose sustenance, livelihoods, and culture have adapted to these dynamic conditions and have simultaneously shaped the fjord systems themselves. Understanding Greenland’s coastal margins and its ecological and human systems (“social-ecological system,” hereafter) therefore requires a “system description” that encompasses the many interacting components responsible for the conditions that presently sustain life.
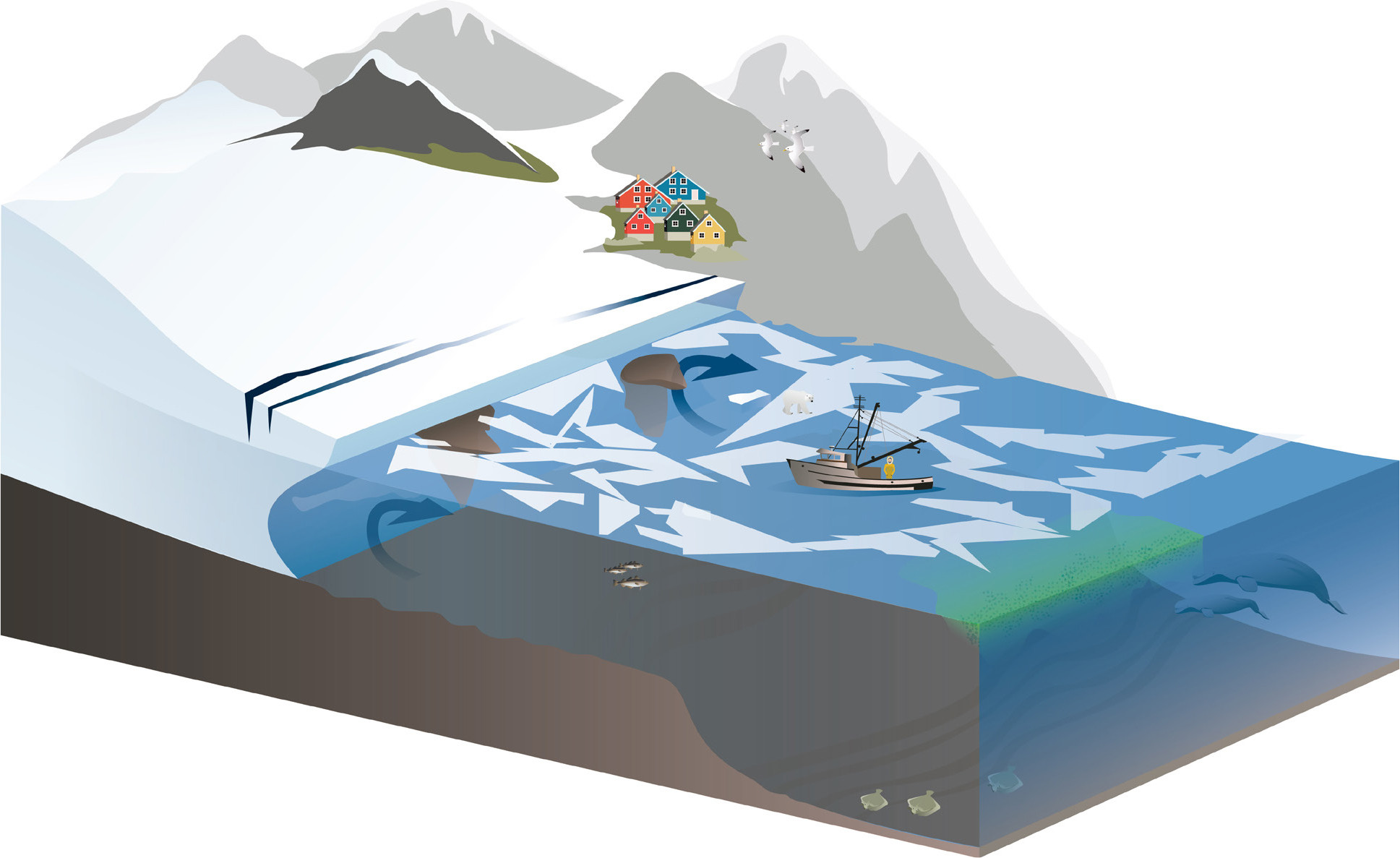
FIGURE 1. Greenland’s coastal margins are influenced by the presence of glaciers and their freshwater and sediment discharges, fjords, sea ice, and currents along the continental shelf, all of which maintain thriving ecosystems and support local communities. Illustration by Jennifer Matthews, Scripps Institution of Oceanography, UCSD. > High res figure
|
Climate change, and the associated pronounced warming of the Arctic (Serreze et al., 2009), is driving rapid change in these regions as manifested by the retreat of glaciers, an increase in surface melt of land-ice, a shortened sea-ice season, rising air temperatures, and a meridional migration of species. Anthropogenically driven change adds to large-scale climatic fluctuations, including those associated with modes of climate variability that naturally characterize this region, such as the Arctic and North Atlantic Oscillations (Hurrell, 1995) and the Atlantic Multidecadal Oscillation (Zhang et al., 2019). Greenland’s resilient human communities have historically adapted to these changes by shifting their hunting and fishing practices (Hastrup, 2018; Nuttall, 2020). However, climate projections indicate that future climate variability will exceed what has been observed over the last century and, eventually, what is recorded in the collective, historical memory of the inhabitants. Identifying when this will occur and understanding how this region will evolve in the future will help inform adaptive strategies for local residents.
In this review, we use data and models to identify the system of environmental conditions around Greenland that relate to indicators of the health of the marine ecosystem, the inshore and offshore fisheries, and the human settlements. One goal is to identify the overarching characteristics that support the thriving coastal environments around Greenland by considering the geographic and climatic forces that shape these environments. Next, we investigate the variability of several system components for two different regions, one in the Atlantic sector and one in the Arctic sector, to determine how variability over the last century might inform changes projected over the next century. The Qaanaaq region of Northwest Greenland and the Ammassalik region of Southeast Greenland are chosen as representative of a range of geographic, climatic, historical, and social settings across Greenland. Historical data and model reconstructions, in particular, are used to identify periods when the climate was similar to that of today and to determine when future projections will emerge beyond historical experience. A follow-up study will further explore the human dimensions of this system to analyze how climate and other biophysical forces intersect over time with political, economic, technological, and cultural forces.
Climate, Ecosystems, Fisheries, and Humans at Greenland’s Coastal Margins – Pan Greenland
Kalaallit Nunaat (Greenland) stretches from the high Arctic (83°N) to the subpolar North Atlantic (59°N; Figure 2). The ~2 km thick Greenland ice sheet covers 81% of its area, confining its 56,000 inhabitants to a thin, ice-free coastal strip along the western and southeastern coasts. About one-third of Greenlanders live in Nuuk, the capital, while the remainder live in communities whose average population is 2,000 (Figure 2f). Greenland’s coastal margins can be partitioned into four different oceanic sectors—the Arctic Ocean, the Nordic Seas, the North Atlantic subpolar gyre, and Baffin Bay—each dominated by different combinations of waters from the Arctic and Atlantic Oceans. Warm, salty Atlantic waters of subtropical origin flow along the eastern and western continental slopes, progressively encroaching onto the shelves through glacially carved canyons (Figure 2c,d). North of Denmark Strait, the Atlantic waters are subsurface and colder as a result of their transformation in the Nordic Seas. Inshore, along the entire eastern coast, the shelf is primarily occupied by cold, fresh water of Arctic origin that is associated with the export of water and sea ice from the Arctic Ocean through Fram Strait (Figure 2c; Kwok et al., 2004; de Steur et al., 2009). Here, sea ice extends south of 60oN, despite the presence of warm waters offshore (Figure 2e). This sea ice, and the associated colder coastal climate, is one of the main reasons that most of Greenland’s inhabitants live on the west coast (Figure 2f), where the influence of the Arctic waters is diminished, and comparatively warm waters fill the continental shelves. Further north, in Baffin Bay, Greenland’s coast is again influenced by Arctic waters, this time flowing south through Nares Strait (Figure 2c). North Greenland borders a cold, mostly sea ice-covered region of the Arctic Ocean characterized by ice ridging and quasi-permanent ice cover. These different water masses affect local air temperatures that are otherwise mostly uniform over much of the island. In particular, during winter, warm, Atlantic waters make the southeast coast warmer than the northwest coast (Figure 2a,b).
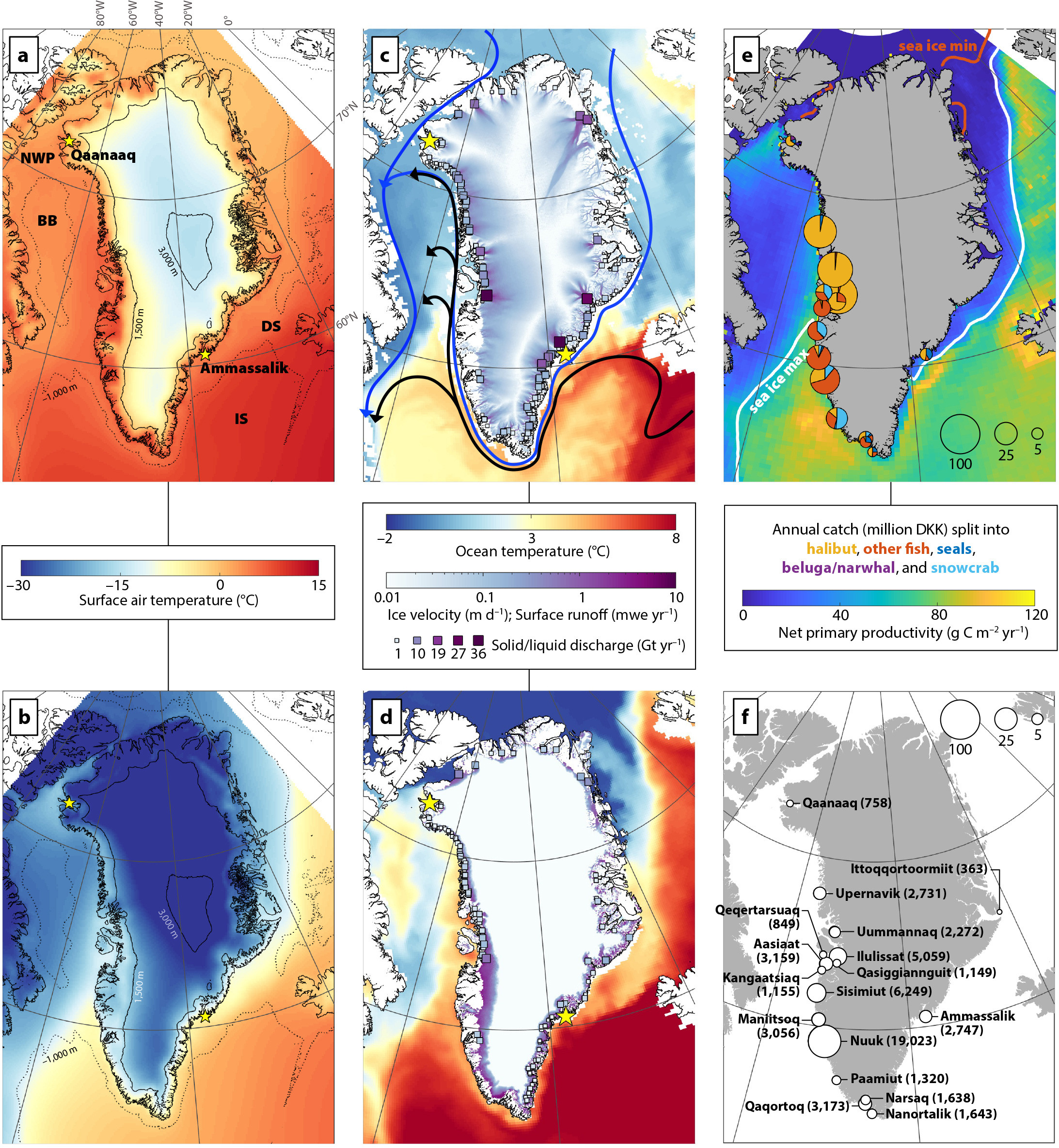
FIGURE 2. Present-day state of aspects of Greenland’s climate, marine ecosystems, and communities. (a) Mean August surface air temperature from ERA5 (Hersbach et al., 2019). DS = Denmark Strait. IS = Irminger Sea. BB = Baffin Bay. NWP = North Water Polynya. (b) Mean February air temperature. (c) Mean August ocean temperature at 200 m depth from the Ocean Reanalysis System 5 (ORAS5; Zuo et al., 2019), ice flow velocity (filled contours; ESA CCI, 2021; mwe = meltwater equivalent), and solid ice discharge from major marine-terminating glaciers (squares, legend below; Mankoff et al., 2019). Arrows show mean currents transporting warm, salty Atlantic water (black) and fresh, cold Arctic water (blue). (d) Mean August surface ocean temperature from ORAS5, mean August surface runoff (filled contours), and the resulting liquid freshwater discharge from marine-terminating glaciers once integrated over their catchments (squares, legend above) from the Regional Atmosphere Climate Model (RACMO; Noël et al., 2018). (e) Annual mean net primary productivity modeled from remote sensing ocean color (Vernet et al., 2021) and annual catch of fish and marine mammals (Statistics Greenland, 2020). (f) Greenland’s primary population centers (inhabitants in 2021; Statistics Greenland, 2020). Panels a, b, and e represent averages over 2003–2018, except for fisheries and marine mammal data, which are averages over 2012–2020. Data in panels c and d are 2018 only, while panel f shows 2021 data. > High res figure
|
Environmental and geographic conditions along Greenland’s margins result in strong spatial gradients in the timing of seasonal phytoplankton blooms and overall rates of primary production (Figure 2e). In general, Greenland shelf waters are medium to low in annual net community production (11–34 Tg C yr–1) relative to other Arctic regions but tend to have higher net integrated primary production when subsurface and under-ice productivity are included in remotely sensed estimates (118 Tg C yr–1; Codispoti et al., 2013; Hill et al., 2013). North-south differences in day length, sea-ice extent, air temperature, and water masses contribute to overall higher rates of production in the south compared to the north (Vernet et al., 2021), with blooms initiated earlier in the south (April versus June), resulting in longer productive seasons. These rates are on par with other productive high-latitude regions (Codispoti et al., 2013; Vernet et al., 2019). In areas that are strongly impacted by the presence of sea ice, including the north and east Greenland coasts (Figure 2e), spatial and temporal variability in primary production is mediated by the timing and mechanism of sea-ice retreat, with blooms typically initiated offshore along the sea-ice margin or under the ice and propagating shoreward (Mayot et al., 2018; Vernet et al., 2021).
Along the sea ice-dominated continental shelves of Northeast and Northwest Greenland, primary production is dominated by two polynyas (annually recurring areas of persistent open water bounded by the coast and pack ice offshore), the Pikialasorsuaq (North Water Polynya) and the Northeast Water Polynya (Tremblay and Smith, 2007; Marchese et al., 2017). The physical processes driving the early seasonal formations and sustained openings of these systems, including wind-driven advection of sea ice offshore, topographic steering and blocking of multi-year ice, and ocean-driven melting of sea ice by Atlantic-sourced waters, also contribute to high supplies of nutrients, allowing for unusually early, prolonged, and productive phytoplankton blooms that support rich marine ecosystems. Fluctuations in the extent of the North Water Polynya, as recorded by the sediment record (Ribeiro et al., 2021), have coincided with major transitions in human occupation of the region, providing evidence for the long-term importance of this polynya to the human-natural coupled system (Speer et al., 2017).
Hundreds of glacial fjords, many containing large, fast-flowing marine-terminating outlet glaciers grounded hundreds of meters below sea level, connect the ocean with the ice sheet. Freshwater from glaciers released into the fjords in the form of icebergs (Figure 2c; Mankoff et al., 2019), meltwater from submarine melting of the ice front (Slater et al., 2019), runoff of surface melt (Figure 2d; e.g., Noël et al., 2016), and basal meltwater (Karlsson et al., 2021) is transformed by processes within the glacial fjords before being exported into the upper layers of continental shelf waters (Beaird et al., 2015, 2018; Moon et al., 2017). Deep troughs that cut across the continental shelf provide a pathway for warm, salty, denser shelf waters to flow into the fjords and drive melting of the glaciers at depth (Jakobsson et al., 2012; Straneo et al., 2012; Snow et al., 2021).
Seasonal surface runoff from the ice sheet’s coastal margins (Figure 2d), discharged at the bases of large glaciers below the ocean surface, triggers rising plumes along the glaciers’ faces, driving mixing and upwelling of deep, nutrient-rich ocean waters (Jenkins, 2011; Slater et al., 2015; Hopwood et al., 2018; Kanna et al., 2018; Cape et al., 2019). High rates of summertime primary productivity and phytoplankton biomass in fjords, coincident with nutrient enrichment of the upper water column downstream of marine-terminating glaciers, have been attributed to this process (Meire et al., 2017). This upwelling of nutrients is also thought to contribute to a lengthening of the growth season within the fjords, with secondary summer blooms accounting for an unusually large fraction of annual primary production (Juul-Pedersen et al., 2015).
Water is continuously exchanged between the fjords and the shelf regions as a result of offshore and katabatic winds, glacial transformation of ocean waters, and other mechanisms (Motyka et al., 2003; Jackson et al., 2014; Moffat, 2014; Carroll et al., 2017; Spall et al., 2017; Fraser and Inall, 2018). Sills and topography mediate this exchange (Mortensen et al. 2014). As a result, marine ecosystems within the fjord and over the continental shelf are not independent. For example, fjord-shelf exchanges have important consequences for the dispersal and retention of planktonic organisms, including larval fish, with consequences for the carrying capacity, recruitment, and genetic exchange among fish populations (Asplin et al., 1999).
The mixing of different water types and the consequent nutrient upwelling renders the shelf and fjord regions productive (Vernet et al., 2021). When the winter sea ice retreats, it triggers large primary production blooms on the shelf that attract high densities of zooplankton and lower trophic level forage fish (Laidre et al., 2008). Boreal forage fish, such as capelin and sand lance, are key species in the food webs of subarctic waters, while Arctic cod play this role in Arctic waters. These fish and zooplankton ultimately attract large numbers of marine mammals and seabirds. Additionally, glacial ice (in the form of the iceberg melange found at the margins of marine-terminating glaciers) provides a year-round habitat that can support Arctic species such as polar bears (Laidre et al., 2022). These unique environmental conditions around Greenland mean that it can host a species assemblage of year-round, true Arctic specialists (polar bears, narwhals, ringed and bearded seals) as well as large numbers of temperate marine mammal species (e.g., subarctic baleen whales, and a suite of dolphins and toothed whales) that migrate to the productive shelf waters during summer.
These rich marine ecosystems also support fisheries (Figure 2e) that are an important economic resource for Greenland, accounting for one-third of its annual revenue and constituting the largest employer after the public sector (Statistics Greenland, 2020). The 200-mile (320 km) fishing territory is composed of two managed fisheries: offshore and coastal (or “inshore,” within 3 nautical miles). The coastal fleet includes more than 1,700 small boats, often owned by individuals, that fish for Greenland halibut, Atlantic cod, snow crab, and lumpfish (Figure 2e). The offshore fleet consists of large fishing vessels owned by corporations that primarily trawl for shrimp and halibut (data not shown). Catches of Greenland halibut, both inshore and offshore, dominate in West Greenland, while catches of cod dominate in East Greenland, especially in areas of Atlantic water influence. While most of the inhabited areas, and all of the fishing processing plants, continue to be in West Greenland, in recent years East Greenland offshore fisheries have yielded most of the annual landings of cod and pelagic species. In addition to fisheries, marine mammal and seabird harvests are important activities.
Historical and Projected Changes in Ammassalik and Qaanaaq
The large spatial variability in climatic conditions around Greenland’s margins, resulting from factors such as proximity to the Arctic or North Atlantic Oceans, the presence of sea ice, freshwater discharge from the ice sheet, glacial discharge, orography, and bathymetry, gives rise to localized areas of high marine productivity (Figure 2e). Human settlements in Greenland are typically located close to a subset of these productive regions where conditions are favorable for hunting, fishing, and human survival. Thus, any analysis of the variability affecting these social-ecological systems must be carried out at a regional scale. Here, we focus on two localities whose characteristics are at different ends of the range of systems in Greenland: Ammassalik in Southeast Greenland and Qaanaaq in Northwest Greenland.
Ammassalik (“the place with the capelin,” located on Figure 2a) comprises six settlements and is one of only two inhabited regions in East Greenland. The largest settlement, Tasiilaq, has 2,000 inhabitants, while the others collectively have fewer than 250. Subjective well-being in this region is influenced by a range of factors, from social and family connections to employment, contact with nature, emotional state, health, education, and other factors that shape social life in East Greenland. In many cases, hunting, fishing, and the collection of local food (e.g., seal, trout, salmon) in and around the fjord system is vital to social well-being and quality of life here (Steenholdt, 2021). Along the coast, warm, salty waters from the Irminger Sea mix with cold, fresh waters from the East Greenland Current and the Coastal Current (Sutherland et al., 2013; Snow et al., 2021) to support high productivity on the continental shelf. In addition, upwelling of nutrient-rich waters from the many tidewater glacial fjords in the region results in an upward nutrient flux that replenishes depleted macronutrients in the upper part of the water column (Cape et al., 2019). These processes sustain a rich and diverse ecosystem that supports fisheries for the Ammassalik communities. Commercial fishing is more limited here than in West Greenland but has nonetheless helped produce economic opportunities, jobs, and infrastructure, such as transportation (Buijs, 2010; Moral García, 2019).
Qaanaaq, with approximately 650 inhabitants, is the largest of three settlements located along Inglefield Fjord in Northwest Greenland (Figure 2a for location). It was established in 1953, when the population from nearby Dundas/Pituffik was forcefully relocated so that the US military could build Thule Air Force Base (Flora et al., 2018; Hastrup, 2019). Inglefield is a wide, deep Arctic fjord where Kangerluarsuup (Bowdoin), Qeqertaarsuusarsuup (Tracy), Qaqujaarsuup (Helprin), and several other smaller glaciers terminate and help create nutrient rich, highly productive waters. Because a series of sills in the northern portion of Baffin Bay partially obstruct the transport of warm Atlantic Water by the West Greenland Current, subsurface waters in Inglefield Fjord are a relatively cold mixture of Arctic and Atlantic Ocean waters (Münchow et al., 2011; Willis et al., 2018). Thus, Inglefield Fjord is expected to be more strongly exposed to Arctic-sourced variability than Ammassalik. The ecosystem is also influenced by the Pikialasorsuaq (the “North Water Polynya”), a large, highly productive polynya in the northern part of Baffin Bay. Qaanaaq’s community has long relied on subsistence hunting for seabirds and marine mammals, including narwhal hunts from kayaks and polar bear hunts from dog sledge trips over the sea ice. Development of a Greenland halibut fishery over the last 10 years within Inglefield Fjord has accompanied other changes in hunting, fishing, and economic opportunities in this coastal region. These activities change not only with sea-ice loss and the shifting ocean-ice interface but also with a rise in tourism and international research that offer cash-based economic opportunities beyond subsistence (Flora et al., 2018).
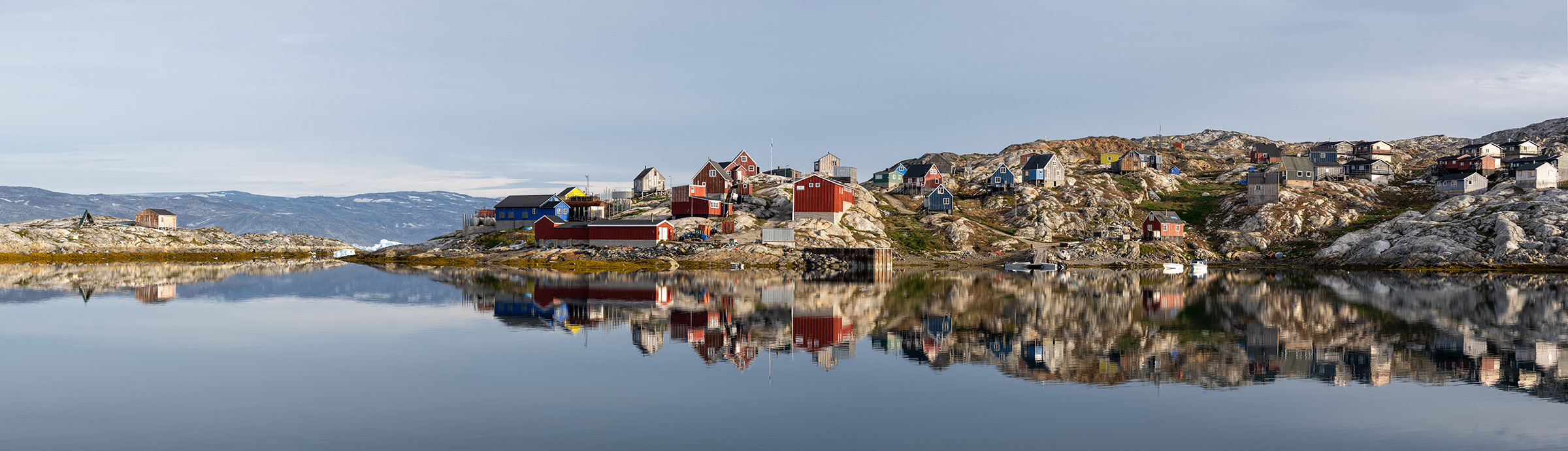
The town of Tiilerilaaq (formerly Tiniteqilaaq) is a small settlement located in the middle of Sermilik Fjord in the Ammassalik district. Its community relies largely on hunting and fishing for sustenance. Photo credit: Jamie Holte, Scripps Institution of Oceanography, UCSD, August 2021. > High res figure
|
Past and Projected Changes in Physical Climate
Historical physical climate data and model reconstructions indicate that Ammassalik and Qaanaaq have exhibited substantial variability in the atmosphere, the ocean, sea ice, and glaciers over the last 120 years (Figure 3), consistent with studies addressing variability on larger scales (e.g., Zweng and Münchow, 2006; Box et al., 2009; Straneo and Heimbach, 2013; Onarheim et al., 2018; Mouginot et al., 2019; Hanna et al., 2021). Collectively, these studies suggest that year-to-year variations in ocean and atmospheric variables over and around Greenland are linked with the North Atlantic and/or Arctic Oscillations (together denoted as NAO; Simpkins, 2021). A negative NAO is associated with a southward shift of the Atlantic storm track, reduced wind stress and wind-stress curl over the subpolar North Atlantic, and a warmer ocean in this region (Häkkinen et al., 2011). Thus, in coastal Greenland, a negative NAO is associated with a rise in coastal air temperatures (especially in West Greenland) and an increase in surface melt (and vice versa for a positive NAO). However, the details vary with each season, and the correlations are strongest in the earliest twentieth century and over the last few decades (Hanna et al., 2013). These same studies suggest that the multi-decadal variations around Greenland (Figure 3) are linked with the Atlantic Multidecadal Oscillation (AMO), where a positive AMO is associated with basin-wide warming of the Atlantic sea surface and air temperatures, and with reductions in sea ice (Hanna et al., 2012; Zhang et al., 2019). Variations in air temperatures in West and Southeast Greenland and subsurface ocean temperatures over the last century, in particular, have been shown to be correlated with the AMO (Hanna et al., 2013; Straneo and Heimbach, 2013). In addition to interannual-to-multidecadal variability, these regions are also exhibiting rising air temperatures and sea-ice reduction associated with the amplification of climate change in the Arctic region (Serreze et al., 2009; Stroeve and Notz, 2018).
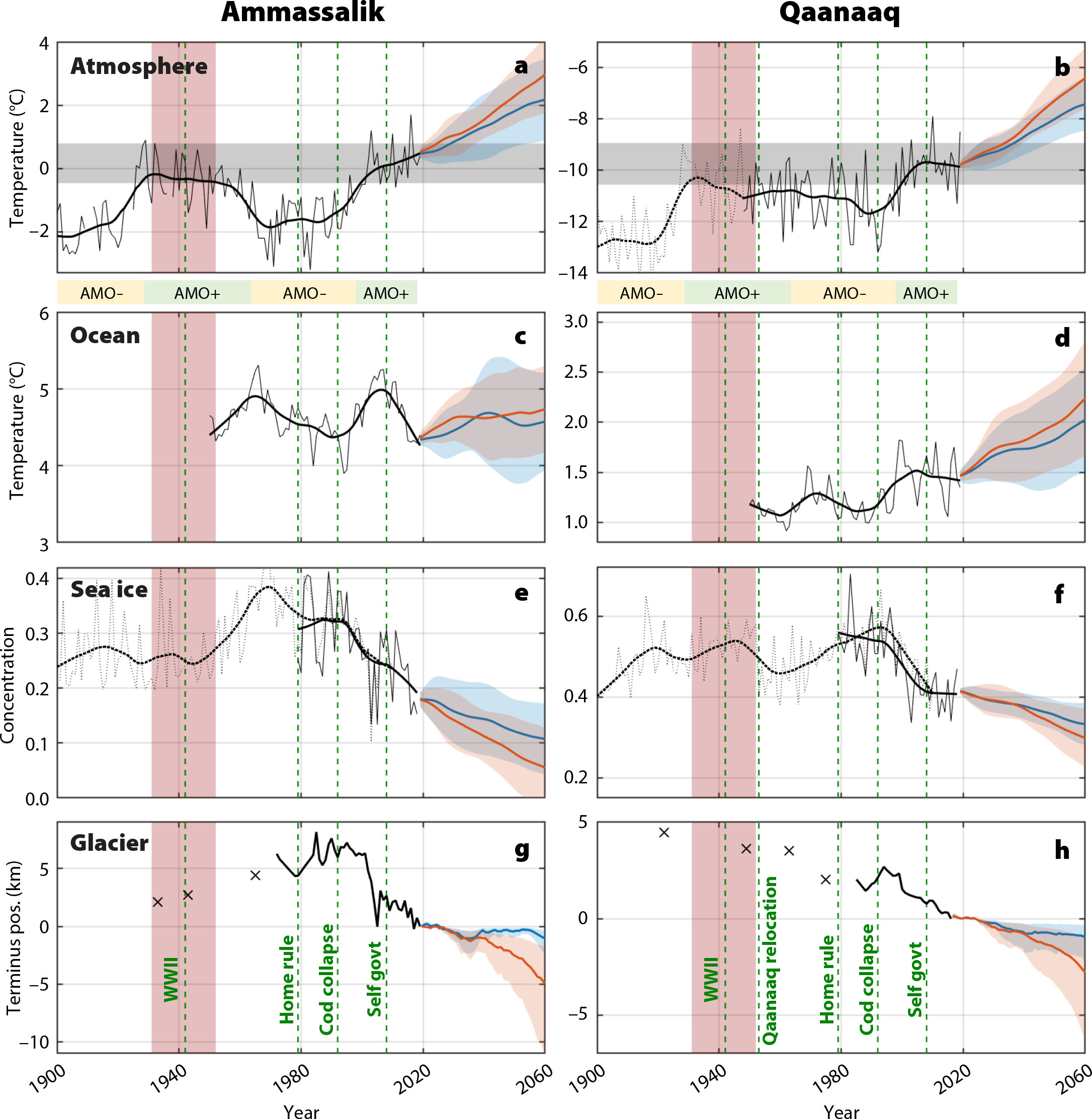
FIGURE 3. Past and projected future variability for the main physical climate components for the social-ecological systems in Ammassalik (left) and Qaanaaq (right), together with examples of societal events relevant to Greenland (green vertical dashed lines). (a, b) Air temperature. Past air temperatures are derived from weather stations (Cappelen et al., 2019), with annual values shown as a thin black line and a 20-year centered mean shown as a thick black line. In Qaanaaq prior to 1948, we use data from nearby Upernavik, shown as dashed lines. The future projections are derived from an ensemble of Coupled Model Intercomparison Project Phase 6 (CMIP6) and show an ensemble mean (thick line) and ensemble standard deviation (shading) for low emission (ssp245, blue; ssp = shared socioeconomic pathways) and high emission (ssp585, red) scenarios. Gray horizontal shading on the air temperature plots shows the observed range over the past 20 years. The red vertical shading on all plots highlights the 1930s and 1940s warm period, while the colored bars beneath the plots show the positive and negative phases of the Atlantic Multidecadal Oscillation (AMO). (c,d) Equivalent for 200–500 m ocean temperature. Past ocean temperatures are derived from the EN4 subsurface temperature and salinity data set (Good et al., 2013) for the Irminger Sea (Ammassalik) and Baffin Bay (Qaanaaq) regions. (e,f) Annual mean sea-ice concentration over regions close to the communities. The solid lines show satellite-derived values (Peng et al., 2013) while the dashed lines come from the Pan-Arctic Ice Ocean Modeling and Assimilation System (PIOMAS)-20C model (Schweiger et al., 2019). (g,h) Glacier terminus positions refer to Helheim Glacier (for Ammassalik) and Heilprin Glacier (for Qaanaaq), with data from Khan et al. (2020) and Sakakibara and Sugiyama (2018). The glacier projections use a terminus retreat parameterization described in Slater et al. (2019). The future projections have had a constant bias subtracted to ensure continuity with recent observations; in this sense, the projections should really be interpreted as anomalies relative to the recent past. > High res figure
|
In terms of ocean properties, we note that the Ammassalik region is dominated by decadal to multi-decadal variability with no discernible long-term trend, while the ocean around Qaanaaq has recently warmed above the range exhibited over the last century (Figure 3). Recent trends in air temperature and sea ice in both regions are discernible when superimposed on the decadal to multi-decadal variability (Figure 3a,b,e,f). A large air temperature warming trend that started in the 1990s (Figure 3a) and diminished beginning in the early 2000s (Hanna et al., 2021) is largely mirrored by a decreasing trend in sea-ice concentration (Figure 3c). Qaanaaq shows a larger reduction in sea-ice concentration than Ammassalik (Cooley et al., 2020), and glaciers in both regions have long been retreating. Helheim began retreating in the mid-2000s following a long period of stability and advance, whereas Heilprin has been retreating continuously since at least the 1920s (Figure 3g,h).
The historical data analysis also shows that present-day warm conditions are comparable to those of the 1930s–1940s (Figure 3), a warm period over the Atlantic and Arctic sectors (Polyakov et al., 2005; Box et al., 2009) that coincided with an AMO positive phase, which has been associated with glacier retreat around Greenland (Bjørk et al., 2012; Khan et al., 2020). The implication is that present-day conditions are not too dissimilar from the conditions that both communities experienced during the 1930s–1940s.
Ensemble projections from climate models indicate that, even in low greenhouse gas emission scenarios, the climate and the cryosphere in both regions are likely to move, within a few decades, beyond the ranges recorded for both communities over the last century (Figure 3). Considering the climate models’ ensemble means, for example, mean annual air temperatures are projected to rise above the past 20 years’ ranges by 2030–2040, and then warm still further by 2060 (Figure 3a,b). The ensemble means also project a reduction in sea ice (Figure 3e,f) and ongoing glacier retreat (Figure 3g,h; Goelzer et al., 2020), while a large spread in the climate model projections for ocean temperature indicates that the future evolution of ocean temperature is uncertain, particularly for Ammassalik (Figure 3c,d). Even considering the spread among climate model simulations, air temperature, sea ice, and glacier terminus position move outside of the range of contemporary human experience by 2060 (Figure 3). Over shorter lead times, the rate of change and emergence of unprecedented conditions will be influenced by the relative amplitudes of internal variability and by forced trends, which vary depending on location and the quantity that is of interest.
Past and Projected Changes in Ecosystems, Fisheries, and Humans
It is expected that projected changes in the physical climate will impact both the shelf areas and the fjords in the Qaanaaq and Ammassalik regions and, in turn, affect the ecosystems and human communities that depend on them (Figure 4). For example, increased subglacial discharge will augment the glacial nutrient pump as long as a glacier is grounded in deep, nutrient-rich waters. Once the glacier retreats into shallower waters, however, the nutrient source for the upper waters of the fjord and shelf is expected to be reduced (Meire et al., 2017; Hopwood et al., 2020). Sea-ice variability will also impact shelf productivity, with longer periods of ice-free conditions supporting higher rates of productivity, as already observed for Baffin Bay and the Arctic Ocean (Frey et al., 2020; York et al., 2020). At present, the productivity data for both regions is limited to a few decades over which significant interannual variability exists but no significant trend emerges (Figure 4a,b). One of the challenges to understanding the evolution of these systems will be identification of the factors governing productivity over longer lead times. Globally, biomass is predicted to decline under all emission scenarios due to increasing temperatures and decreasing global primary production (Lotze et al., 2019); Greenland and other high-latitude regions, however, are expected to respond differently (Wassmann and Registad, 2011; Oksman et al., 2022).
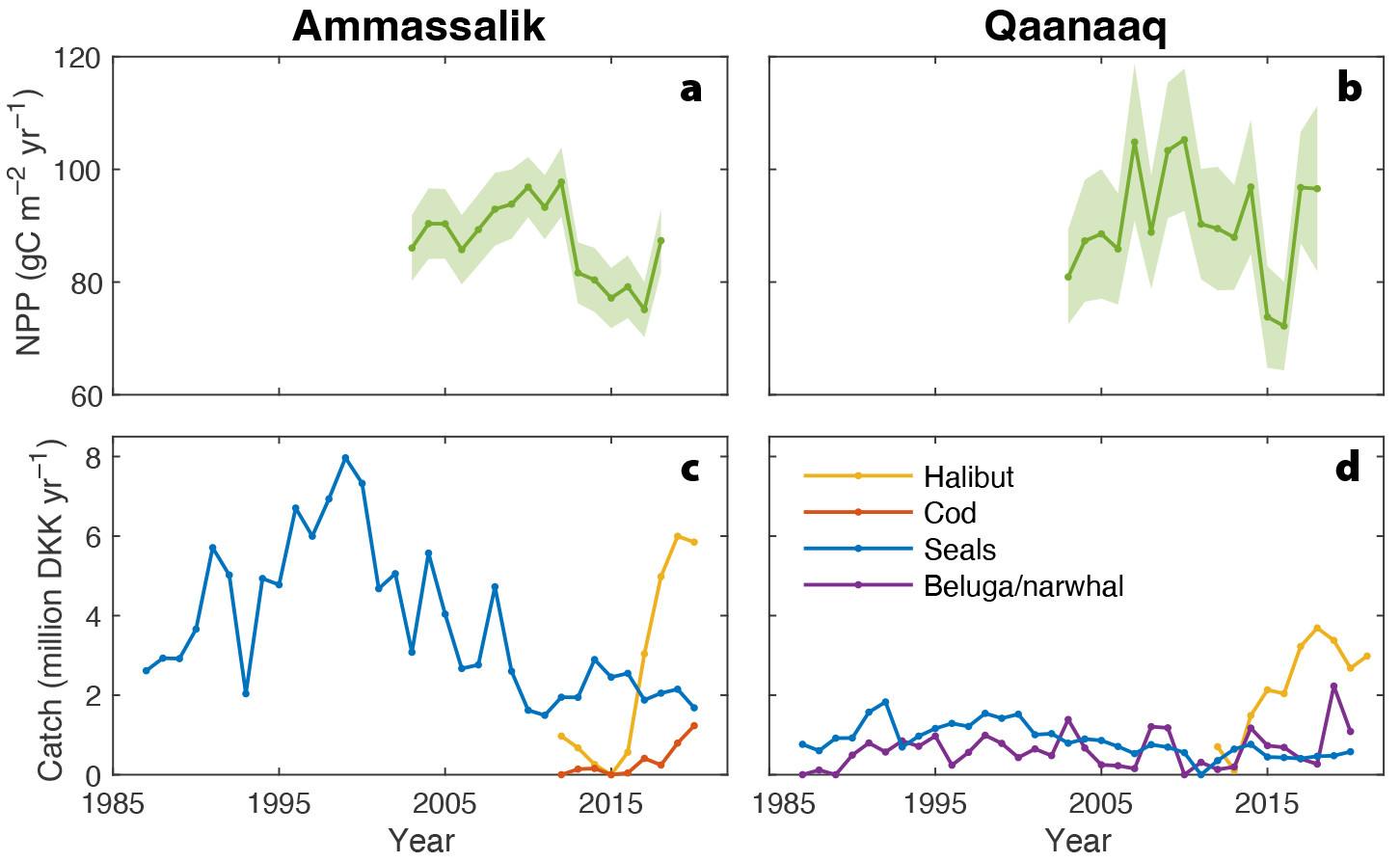
FIGURE 4. Interannual variability in net primary productivity (NPP), fish catch, and marine mammal hunting (a,b). Annual primary production ± standard deviation of monthly means is plotted in gC m–2 yr–1 on the shelf and shelf break. Data are based on weekly averages of multi-sensor satellite ocean color composites (as in Vernet et al., 2021) for the (a) Ammassalik and (b) Qaanaaq (including the North Water Polynya) regions. Note similarity in rates at the two locations separated by 15° of latitude. Fish catch and hunting of seals and beluga/narwhal are shown in millions of Danish kroner per year in inshore waters of (c) Ammassalik and (d) Qaanaaq (Statistics Greenland, 2020). > High res figure
|
The existing fisheries records of catch for the two regions are too short to identify clear trends or investigate how their variability relates to shifting environmental conditions over the last few decades (Figure 4c,d). In the future, further insight may be gained through co-production of knowledge that integrates the memory of the community with knowledge of climate variability. Here, we summarize what is known and include several considerations that highlight some of the mechanisms that can influence fisheries variability.
The Greenland fishing industry, particularly on the east coast, is poised to grow in the coming years as some of the distribution ranges of commercially harvested species continue to shift northward into newly productive waters. In Southeast Greenland, for example, the value of Greenland halibut commercial fisheries now accounts for the largest part of the economy (47% on average between 2012 and 2020), surpassing ringed seal (31%) and harp seal (11%), which were the most important species in the past in terms of coastal resources (Figure 4c). This is also evident in the last decade with the increase in catch of pelagic species offshore, such as capelin, blue whiting, herring, and mackerel. Cod catches in East Greenland are also expected to increase due to new spawning aggregations identified at Kleine Bank (offshore of Ammassalik) and greater transport and migration of cod eggs, larvae, and juveniles from Icelandic waters. As a result, Tasiilaq is being considered as the location for a new processing industry. However, it is unclear how new offshore fisheries (e.g., mackerel and other pelagic fish) or existing ones (cod) will evolve.
Qaanaaq and Ammassalik differ from the rest of Greenland in that marine mammal harvesting is very important for food security, culture, and the local economy in these two communities (Figures 2e and 4c,d). In Qaanaaq, traditional and sustenance activities (marine mammal hunting, winter ice fishing for Greenland halibut, and seabird harvesting) will most likely decline with climate change for numerous reasons, among them ice cover limiting access to ice-associated activities and species, shifts in zooplankton assemblages from dominance of larger Arctic species (e.g., Calanus glacialis, Calanus hyperboreus) to smaller subarctic/boreal species (e.g., Calanus finmarchicus; Møller and Nielsen, 2020), and replacement of Arctic cod by forage fish of boreal origin such as capelin and sand lance. These changes carry the potential to impact the food security and economy of local communities and should therefore be closely monitored and considered in community planning exercises. Although increased opportunities for commercial fishing for Greenland halibut may improve employment and the economy in these communities, there is much uncertainty about the processes that regulate distribution and abundance of these species. For example, Greenland halibut in Upernavik, Uummannaq, and Disko Bay areas show signs of overfishing, as evidenced by a decrease in the mean length of the landed fish (NAFO, 2020). These various points illustrate not only the potential impacts of climate change on fisheries but also the ways in which government regulations, available knowledge, markets, fishing technologies and practices, and environmental conditions all intersect to influence communities, fisheries, and the coastal social-ecological systems.
Discussion
The confluence of Arctic and Atlantic currents, synoptic wind events, glaciers, and sea ice creates conditions that foster healthy and rich ecosystems in Greenland’s glacial fjords and continental shelf waters. Processes such as the topographically induced mixing and upwelling at glaciers’ termini enrich the surface waters with nutrients. Seasonal sea-ice melt sets the timing for the phytoplankton blooms that characterize these regions. Greenland’s large latitudinal range, spanning Arctic and subarctic regions, results in large spatial variations in climate, land and marine-terminating glaciers, and sea-ice cover. Greenland’s coastal margins are characterized by diverse ecosystems that support polar and subarctic species. The locations of settlements around Greenland are, to a large extent, influenced by the availability of these marine resources.
Climate change is rapidly affecting the different processes that foster healthy ecosystems around Greenland. The two locations we focus on here have distinct climates. Ammassalik is strongly influenced by the Atlantic Ocean, with mild winters and a long sea ice-free season. Qaanaaq is Arctic in character, with long cold winters and a short sea ice-free season. Yet, both are affected by substantial multidecadal and interannual variability in air and ocean temperatures and sea-ice conditions. The contemporary climate has some precedent within recent human memory due to a warm period during the 1930s to 1940s, but by the middle of the twenty-first century the climate and cryosphere will almost certainly move beyond contemporary experience. Predicting precisely how the physical climate will evolve in the coming decades, and its impact on the cryosphere, ecosystems, and human communities, remains challenging for a number of reasons. Chief among them is the coupling between systems; for example, a retreat and shoaling of marine-terminating glaciers (itself challenging to predict) may impact marine ecosystems and fisheries through reduced nutrient upwelling, but such links are poorly understood. Some insight may be gained from assessing how past variability has played a role in any ecosystem or human system change. However, this study shows that while we have enough data to reconstruct the regional, physical climate variability over the last century and—assuming an understanding of linkages—how this may have resulted in conditions that support life at Greenland’s margins—there are not enough data to reconstruct the ecosystem variability. Key to future understanding, therefore, is the collection of long-term local and regional ecosystem and fisheries data and the integration, and co-production, of knowledge with local communities. For future projections, there are also issues of scale; the climate models used for projection do not resolve the processes and details of Greenland’s coastline, and it therefore remains unclear how well these models reflect local conditions. Furthermore, collaborative approaches are needed to investigate the evolution of the social-ecological systems that include hunters and fishers (e.g., Planque et al., 2019; Mathis et al., 2015).
The social-ecological system at Greenland’s margins is changing in response not only to global climate change but also to other processes, with varying impacts across the country. Tourism and scientific research in Greenland increasingly bring outsiders to the island, benefiting some through new jobs but also disrupting others—docking of cruise ships and accompanying infrastructure may favor tourists rather than residents. In recent decades, new government regulations have, in some cases, had more profound impacts on local hunting and fishing than has climate change. At the same time, sea-ice loss can sometimes allow greater access to harbors and open up new areas to lucrative mining, while in other cases thinning sea ice thwarts hunting, travel, and transportation. Changes in the social-ecological systems are uneven and inconsistently distributed across the Greenland population. They need to be understood in their own contexts (local, national, global) and by recognizing interacting human and biophysical processes. The development of participatory scenarios with multiple stakeholders (hunters, fishers, natural scientists, social scientists, policymakers) to anticipate future changes in these marine social-ecological systems will be key to providing contextual information for adaptive planning.
Acknowledgments
This work was supported by a planning award (1928007) and an implementation award (2127241) from NSF’s Navigating the New Arctic program. DS acknowledges NERC Independent Research Fellowship NE/T011920/1.