Introduction
The waters of the Alaskan Arctic (here defined as the interconnected US sub-regions of the northern Bering, Chukchi, and Beaufort Seas) are undergoing rapid and profound environmental and ecological changes due to substantial decreases in sea ice quality, extent, and duration as a result of atmospheric and ocean warming. Additionally, with a predominantly northward flow of water through the Bering Strait, alterations in southern and northern Bering Sea marine ecosystems are now propagating into the Chukchi Sea and beyond (Huntington et al., 2020), leading to cascading effects on marine ecosystems (Stevenson and Lauth, 2019). Among other warming-related impacts, harmful algal blooms (HABs) are emerging as a threat to marine-dependent species in the region, including humans. Although HAB species were first documented in the Alaskan Arctic as early as the mid-twentieth century (Bursa, 1963), new evidence suggests that their occurrence and future impacts may be much more widespread and severe than previously thought (Anderson et al., 2021a).
HABs are proliferations of algae that cause harm in a variety of ways, with a key mechanism being the production of potent toxins responsible for illness and death in humans and wildlife (Anderson et al., 2012). In the Alaskan Arctic, like elsewhere in the world, toxic algae directly enter the marine food web through planktivorous filter feeders, such as clams and zooplankton, and can accumulate to levels that sicken or kill higher trophic level consumers, including humans (Figure 1). Shellfish have historically been considered the primary source of dietary exposure for toxins, but a significant difference in the Alaskan Arctic is that coastal residents rely on a large diversity of marine resources for food, adding a new and poorly understood dimension to the threat from HABs.
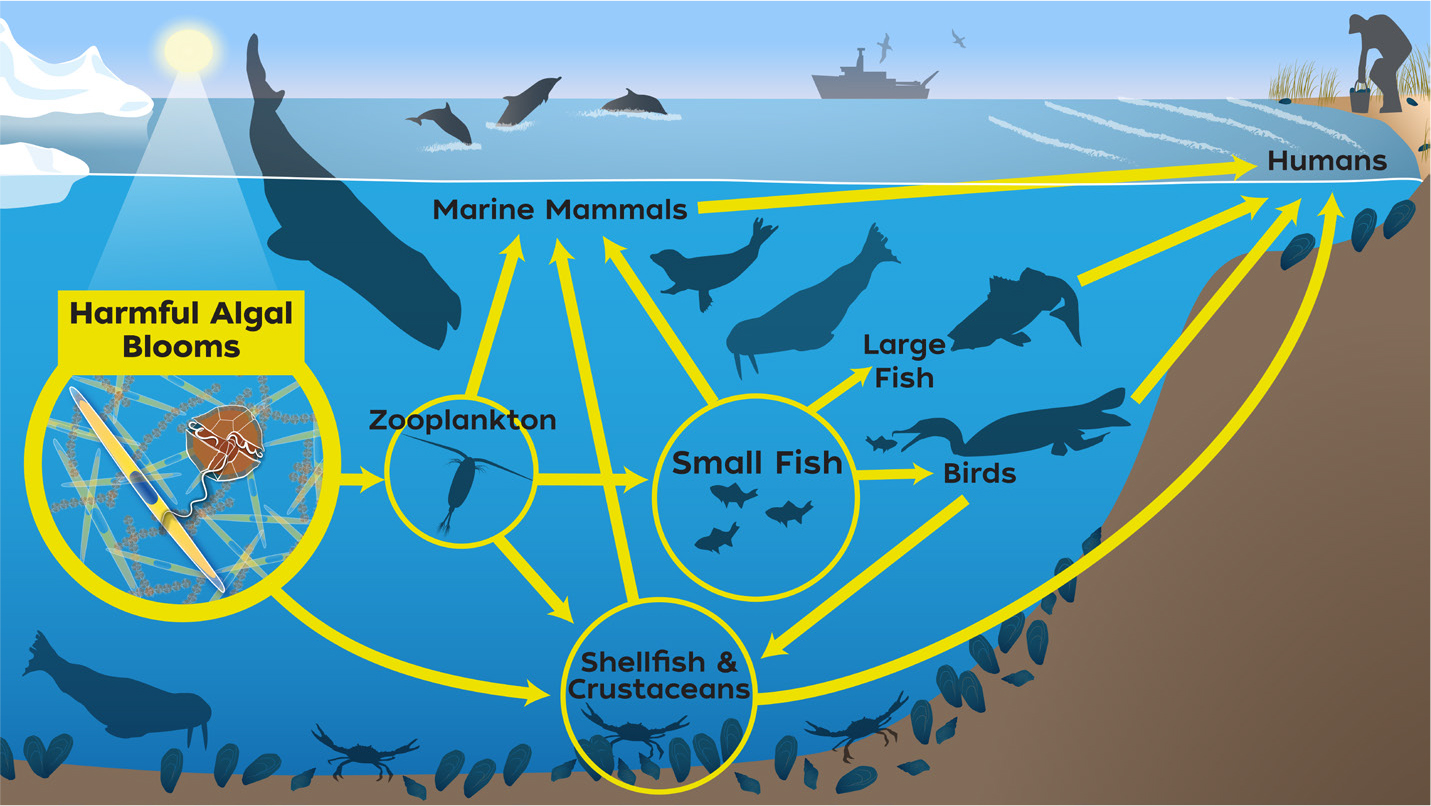
FIGURE 1. Toxins produced by harmful algal blooms (HABs) can be accumulated and transferred throughout the food web when algal cells are eaten by zooplankton, fish, and shellfish that are, in turn, consumed by other animals and humans. At sufficiently high levels, these toxins can sicken or kill both humans and wildlife. Illustration created by Natalie Renier, WHOI Graphic Services. > High res figure
|
There are two primary HAB toxins of concern in this region: (1) saxitoxin and its congeners (hereafter, STXs) produced by the dinoflagellate species Alexandrium catenella, and (2) domoic acid (DA) produced by some diatom species in the genus Pseudo-nitzschia. In many areas of the world, these toxins cause paralytic and amnesic shellfish poisoning (PSP and ASP, respectively) when shellfish are the toxin vectors, but both can also accumulate in other marine organisms through food web transfer (Figure 1). DAP is the term used to describe domoic acid poisoning among wildlife. Other HAB toxins are likely present within the region as well (e.g., diarrhetic shellfish toxins [DSTs] produced by Dinophysis spp.), but these are not presently viewed as significant threats.
Recently, STXs and DA have been detected in marine species throughout the Alaskan Arctic at a variety of trophic levels, including in benthic invertebrates, zooplankton, forage fish, seabirds, and marine mammals (Lefebvre et al., 2016; Van Hemert et al., 2021a). In most cases, reported concentrations in marine wildlife have been relatively low, but potential acute and chronic effects on wildlife health require further investigation. Likewise, there are no recent medical reports of impacts on human health, but the presence of HAB toxins across multiple trophic levels that serve as human food resources, combined with current and projected impacts of climate change (Anderson et al., 2021a), suggest a growing risk that warrants additional research and action.
Much of the Alaskan Arctic faces unique obstacles in monitoring and responding to HABs due to difficult logistical access and lack of response infrastructure. Besides concerns about food safety due to accumulation of toxins in marine organisms consumed by humans, HABs can impact food security by affecting fish and wildlife populations directly (i.e., causing illness or death among animals), further limiting access to these resources. The dearth of current knowledge about HABs in the Alaskan Arctic underscores the need for expanded research, monitoring, education, and communication to address food security, conservation, and public/wildlife health concerns. In this review, we summarize the primary HAB threats to the Alaskan Arctic, identify potential sources of exposure in the marine food web, and discuss implications for human and ecosystem health along with challenges to HAB monitoring and management in this dynamic and rapidly changing environment.
Alexandrium and STXs
Currently, the most significant threat to human and ecosystem health from HABs in the Alaskan Arctic is from Alexandrium catenella, a cyst-forming dinoflagellate that produces STXs. These toxins can accumulate in fish or shellfish to levels sufficient to cause illness and death in human consumers, as well as mortalities of marine mammals, birds, and fish. STXs have long been a problem in the Gulf of Alaska, with reports of illness and fatalities in southeastern and south-central Alaska dating back more than 200 years (Lewitus et al., 2012). In contrast, there are few documented reports in the Alaskan Arctic, though Indigenous oral history cited by Fair and Ningeulook (1995) describe “a red tide at one time which caused many deaths” at Ipnauraq (located in the US Bering Strait region), though no details were provided on the food consumed, symptomatology, or when this occurred.
Alexandrium catenella has a unique multi-stage, meroplanktonic life cycle that allows it to survive unfavorable conditions in seafloor sediments and bloom seasonally in surface waters. While planktonic blooms and shellfish toxicity are predominantly caused by vegetative (swimming, photosynthetic) cells, this species also produces a resting cyst that lies inactive on or near the seafloor and germinates when temperatures and other conditions are favorable. The distribution and density of resting cysts are used to predict the location and timing of future bloom occurrences in some regions, such as the Gulf of Maine (Anderson et al., 2014). Alexandrium blooms generally occur in the spring at temperate latitudes but appear to be present in the late summer and into early fall in the Arctic (Anderson et al., 2021a).
In coastal regions north of the Bering Strait, observations of A. catenella are limited to a few sporadic reports over many years, and blooms have historically not been a significant food safety concern. However, changing environmental conditions driven by warming ocean temperatures are providing an increasingly hospitable environment for A. catenella growth and persistence.
Multiple observations by several research groups over the past decade have provided clear evidence of widespread and dense Alexandrium cyst and cell concentrations in the Alaskan Arctic, indicating the potential for significant bloom development in waters where temperatures were formerly unfavorable. Gu et al. (2013) were the first to identify A. catenella in the US portion of the Chukchi Sea (hereafter simply termed “Chukchi Sea”) and report the toxicity of several isolates. Natsuike et al. (2013, 2017) subsequently reported high concentrations of A. catenella resting cysts in sediments on the Chukchi shelf, as well as bloom populations in the water column, and suggested that the cells were transported northward from the northern Bering Sea. Recently, extremely high concentrations of Alexandrium cysts and vegetative cells were documented over large areas in the Chukchi Sea and adjacent waters, and over multiple sampling years (Anderson et al., 2021a). These surveys reveal a massive and persistent cyst accumulation zone (cystbed) on the seafloor of the Chukchi Sea, extending westward to (and presumably beyond) the maritime border between the United States and the Russian Federation (Figure 2). Maximum cyst concentrations in this cystbed are among the highest reported for this species globally. Bloom populations of A. catenella documented in surface waters of the Bering Strait and the Chukchi Sea were also notable, with dangerously high cell concentrations covering very large areas. As with the Chukchi Sea cystbed, these planktonic blooms were certainly more widespread than were sampled, extending an unknown distance into Russian waters where sampling was not possible. The A. catenella cystbed in the Chukchi Sea is the largest in extent and overall abundance globally. It is at least six times larger in area and 15 times greater in cyst abundance compared to a similar feature in the Gulf of Maine (Figure 2 inset) that sustains large-scale, annually recurrent, and dangerous blooms (Anderson et al., 2014). The same now seems likely in the Alaskan Arctic.
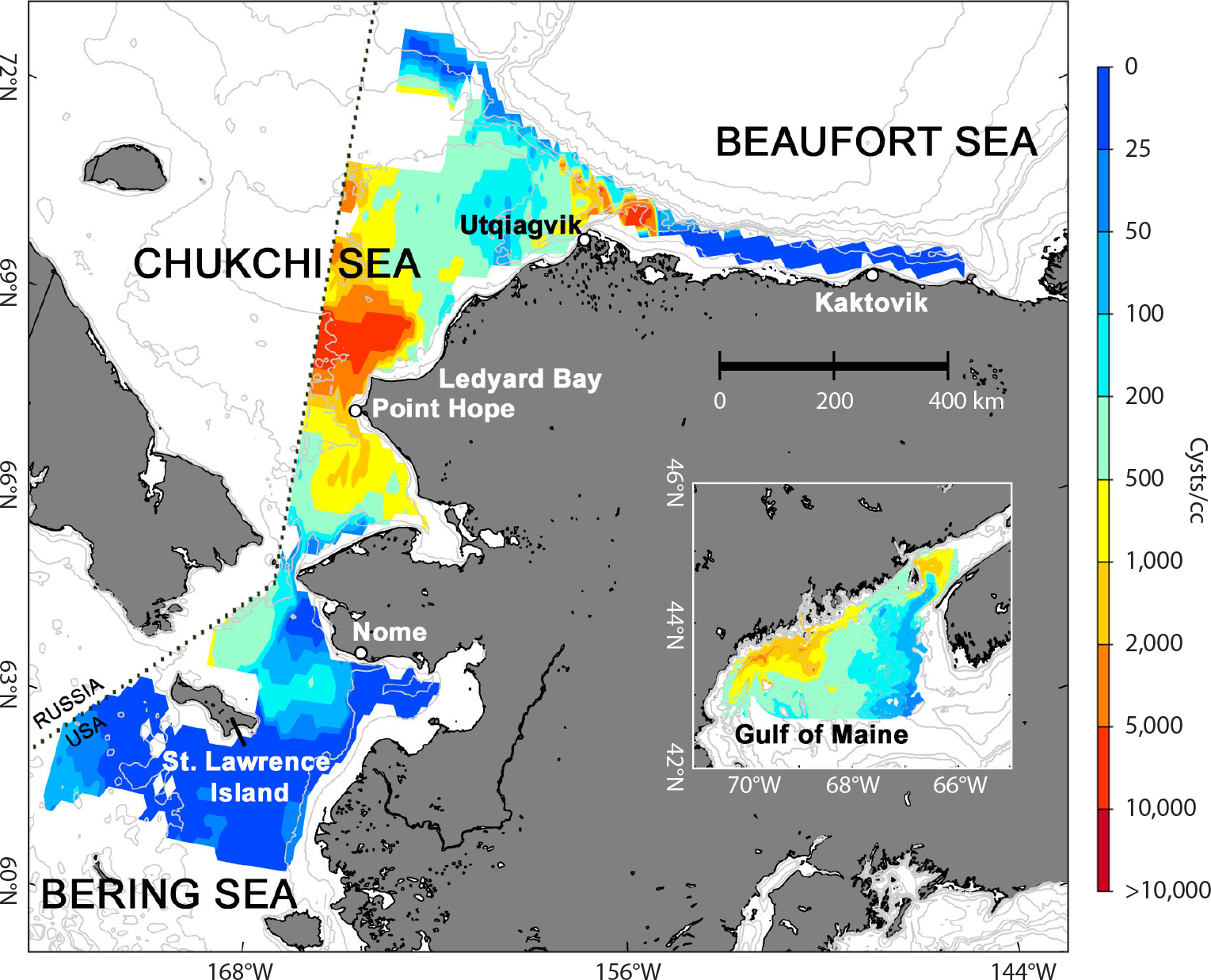
FIGURE 2. Alaskan (2018–2020) and Gulf of Maine (2004–2012) Alexandrium catenella cyst abundance in surface sediments, depicted on the same scale (Albers Equal-Area Conic projection). Sites visited across multiple years were averaged to create these composite maps. > High res figure
|
The origins and development of these Arctic blooms as well as the formation and persistence of the regional cystbed can be attributed to two mechanisms (Anderson et al., 2021a). The first involves northward transport of A. catenella populations through the Bering Strait into the Chukchi Sea from established blooms in US and Russian waters to the south (Figure 3a), as originally proposed by Natsuike et al. (2017). North of Cape Lisburne on the Chukchi Sea shelf, the poleward flow weakens due to the gentler bottom slope, allowing cysts to settle and accumulate; a similar mechanism occurs on the Alaskan Beaufort Sea shelf just east of Point Barrow where another cystbed is located (Anderson et al., 2021a). Over many years, this slowing of the circulation, coupled with episodic advection of southern blooms with resulting cyst production and deposition, has created a regional cystbed of unprecedented size and density. Importantly, historic ocean seafloor temperatures in this cystbed region were likely too cold to support significant cyst germination, with most cysts cycling repeatedly between dormancy (alive but unable to germinate) and quiescence (able to germinate but waiting for favorable conditions) (Fischer et al., 2018). In this scenario, most cysts would remain in the seafloor sediments, unable to germinate and become active because of cold temperatures, with repeated deposition events from transported blooms exceeding small germination losses (Figure 3b). Alexandrium can survive as a cyst for a century or more (Miyazono et al., 2012) until conditions are appropriate for growth. This imbalance between inputs and losses and the longevity of cysts may explain the extraordinary size and density of the Alaskan A. catenella cystbed.
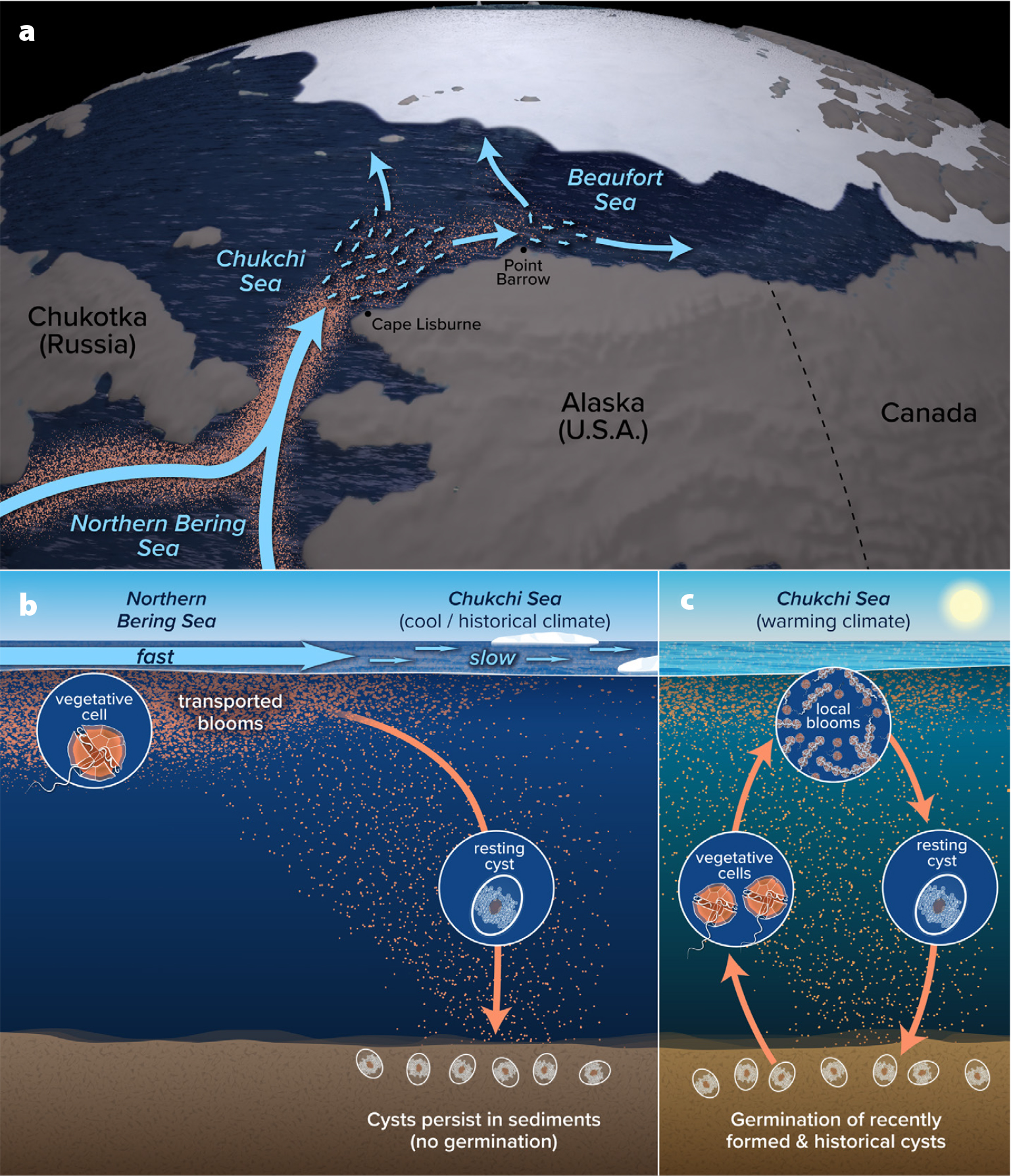
FIGURE 3. Schematic diagrams of Alexandrium catenella bloom dynamics in the Alaskan Arctic region. Panel (a) depicts the transport of blooms (orange dots) from the northern Bering Sea into the Chukchi Sea and beyond. North of Cape Lisburne and again east of Point Barrow, flow speeds decrease (represented by the smaller arrows), allowing Alexandrium cysts to be deposited (b). Panels (b) and (c) show two scenarios for bloom and cyst dynamics. (b) Bottom waters were historically too cold to promote germination of cysts, which presumably cycled repeatedly through dormancy and quiescence. Meanwhile, continued deposition of new cysts occurred via blooms transported from the south. Such sustained inputs led to extremely dense cyst concentrations and a large cystbed. (c) With warmer bottom water temperatures, cysts are able to germinate and initiate local blooms that in turn deposit new cysts to sustain the process. These locally formed cysts are supplemented with those produced by transported blooms, again leading to large and dense cystbeds. Graphics created by Natalie Renier, WHOI Graphic Services. > High res figure
|
Historically, the main threat to wildlife and human health from STXs was from episodic, advected blooms in the waters overlying the “sleeping giant” Chukchi Sea cystbed (Figure 2; Anderson et al., 2021a). Now, however, rapid warming of the bottom waters of the Chukchi shelf has exceeded the temperature threshold above which substantial cyst germination and vegetative cell growth can occur. The warming is due to increased heat flux through the Bering Strait, which is driven by the greater heat content of northern Bering shelf waters, together with enhanced northward volume transport. The former is due to stronger atmospheric heating, and the latter results from a larger sea level height difference between the Pacific and the Arctic.
This changing thermal regime favors a second mechanism of bloom development: local bloom initiation from the Alaskan Arctic cystbed (Figure 3c). Anderson et al. (2021a) estimate that the approximately 2°–4°C increase in bottom water temperatures in the Chukchi Sea over the past two decades has likely increased cyst germination flux twofold and advanced the timing of cell inoculation into the euphotic zone by 20 days. Furthermore, warming of surface waters supports more rapid cell division and bloom development, as well as prolonged bloom duration. Together, these complementary mechanisms of bloom development in the region, along with continued warming, dramatically enhance the potential for large-scale, self-initiating, and annually recurrent blooms, with more intense and widespread HAB impacts.
Pseudo-nitzschia and DA
In recent decades, DA produced by the pennate diatom genus Pseudo-nitzschia has emerged as a serious threat in coastal waters of North America, causing ASP and DAP events and fisheries closures on the Atlantic, Pacific, and Gulf of Mexico coasts and across a wide range of latitudes (Anderson et al., 2021b). While the Alaskan Arctic has not experienced DA events at the magnitude observed at lower latitudes, the presence of DA in Arctic phytoplankton and macrofauna points to an emerging threat (Lefebvre et al., 2016; Huntington et al., 2020). With Pseudo-nitzschia blooms and DA production linked in part to anomalously warm ocean conditions in the northeast Pacific (McKibben et al., 2017), such as warm phases of the Pacific Decadal Oscillation and the Oceanic Niño Index, more severe DA events could occur due to Arctic warming.
With over 50 described Pseudo-nitzschia species, this genus occupies a wide range of temperature and salinity regimes, from estuarine to open-ocean to sea ice (reviewed by Bates et al., 2018). Approximately half these species produce DA, but to varying degrees. Cryptic morphological diversity among Pseudo-nitzschia species further complicates efforts to monitor and understand the ecological conditions that result in DA production. Data on the diversity and distribution of Pseudo-nitzschia assemblages in the Alaskan Arctic are limited, but at least six species of predominantly polar, subpolar, or temperate origin have been reported, many of which occur in sea ice (Figure 4; Poulin et al., 2011; Percopo et al., 2016). Several produce DA in culture, including P. seriata and P. obtusa, although few Arctic strains have been cultivated and tested (Bates et al., 2018; Weber et al., 2021).
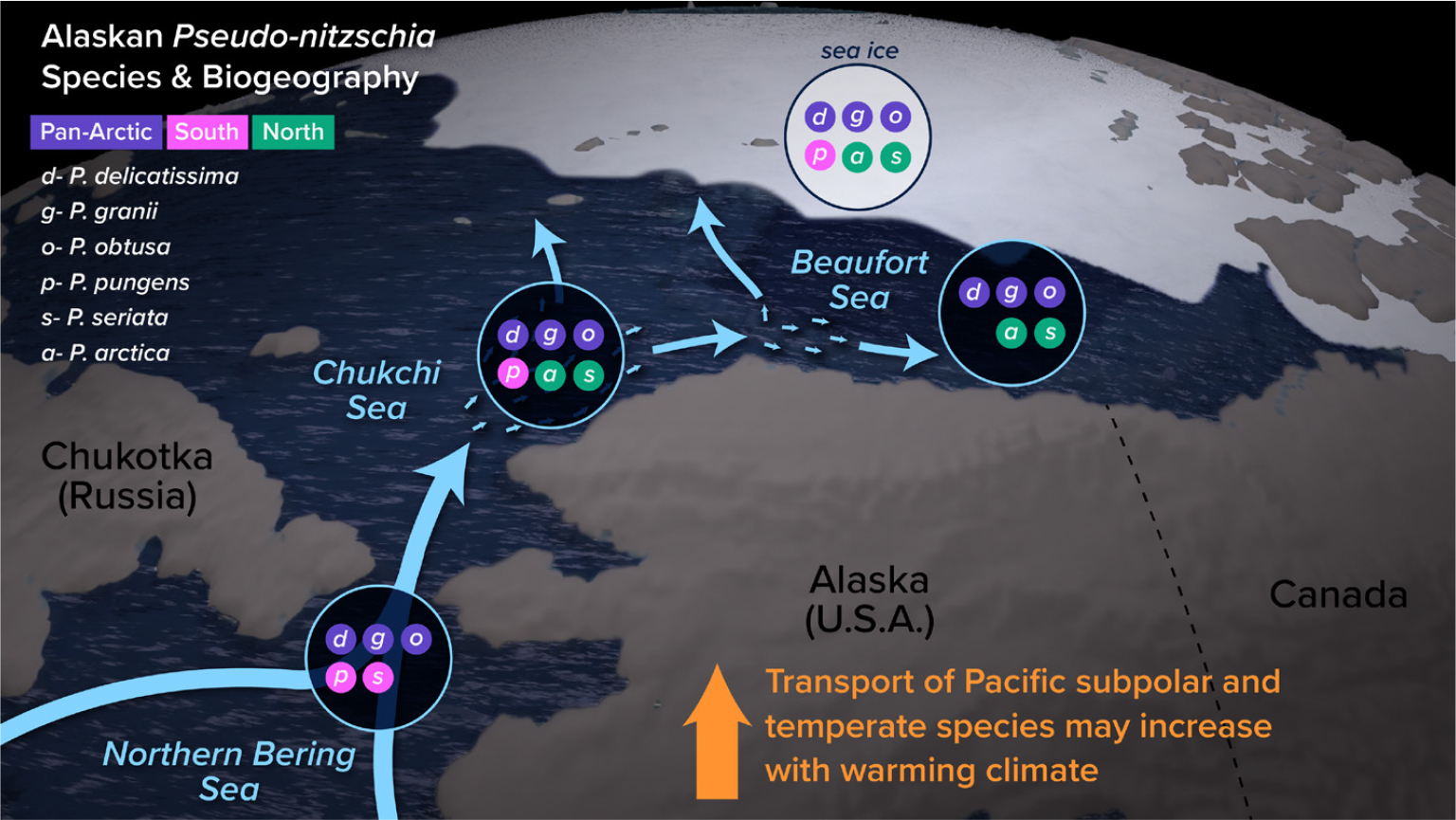
FIGURE 4. Distribution of Pseudo-nitzschia species reported to occur in the Alaskan Arctic (Bering Sea, Chukchi Sea, Beaufort Sea, and sea ice; Bates et al., 2018; recent work of author Hubbard and colleagues). Species are color-coded based on biogeography in the Alaskan Arctic, including distribution across all subregions shown (Pan-Arctic), those suspected to have a more southern origin (in pink), and those suspected to have a more northern origin (in green). Specific locations were not referenced for P. pseudodelicatissima, which may have been confounded with the recently described P. arctica (Percopo et al., 2016). For P. seriata, two genetically distinct populations are shown: one previously observed in temperate Pacific waters, and one observed in Atlantic Arctic waters. Illustration created by Natalie Renier, WHOI Graphic Services. > High res figure
|
For toxic species, biological, chemical, and physical factors such as temperature, light, nutrient availability, sexual reproduction cycles, and the presence of grazers can influence DA production (Bates et al., 2018). Arctic isolates of P. obtusa and P. seriata increased DA production after exposure to copepods: P. seriata increased production by 3300% and toxicity was induced in P. obtusa, previously considered non-toxic (Harðardóttir et al., 2015). This was most likely a chemically mediated reaction, because DA production in P. seriata also increased following exposure to copepod exudates (Tammilehto et al., 2015). These findings suggest that co-abundance of Pseudo-nitzschia species and zooplankton (which also concentrate DA), in addition to other factors, should be considered when evaluating DA toxicity in the Alaskan Arctic.
The majority of DA observations in the region are associated with marine wildlife rather than plankton, but recently, toxin production by plankton assemblages has been observed. During the summers of 2017 and 2018, DA was detected in seawater collected from the northern Bering Sea, Bering Strait, Chukchi Sea, and Beaufort Sea (Huntington et al., 2020; recent work of author Hubbard and colleagues), suggestive of broad regional distribution of toxic species. Particulate concentrations of DA measured in the Alaskan Arctic were generally low (<311 ng L–1; Huntington et al., 2020) compared to the high levels (15,000 ng L–1) that can occur in the US Pacific Northwest (McCabe et al., 2016), a region with recurring DAP events that is connected to the Alaskan Arctic by major current systems. Duration of blooms in the region is currently unknown (and a bloom threshold has yet to be operationally defined for the Alaskan Arctic), but the persistence of cells in sea ice and limited observations from seawater samples, as well as DA in biota, suggest that Pseudo-nitzschia is likely present year-round.
In other regions, Pseudo-nitzschia species composition, abundance, and DA concentrations are known to change rapidly over time and space due to ocean currents and changing ecological conditions. This is the case in the Alaskan Arctic as well, where varied water masses and dynamic circulation are important determinants in the distribution of Pseudo-nitzschia spp. and zooplankton grazers over short and long timescales. Indeed, a diversity of species occur across the region and over seasons, including in sea ice (Poulin et al., 2011; Bates et al., 2018). Given the warming temperatures of Pacific-origin water entering the Arctic through the Bering Strait, together with the increased flux of this water, there is the potential for northward expansion of more temperate and subpolar Pseudo-nitzschia species (Figure 4). Predicted changes in hydrographic regimes and sea ice extent/duration, coupled with complex and environmentally dependent mechanisms underlying growth and DA production, suggest that multiple factors are likely to be important for toxicity over varying timescales.
Although there is still much to be learned about the potential risk to human communities and wildlife populations in the Alaskan Arctic from this important HAB group, the presence of toxic Pseudo-nitzschia species indicates potential for trophic transfer through Alaskan food webs. Fortunately, DA levels detected thus far in water and wildlife (see below) have been low, but certainly warrant continued research and monitoring. The potential effect of DA on Arctic ecosystems is a major concern, though not enough is known of lethal doses and toxin transfer pathways to be definitive at this time. Likewise, predicting what projected ocean warming will do to distribution and abundance of toxigenic Pseudo-nitzschia species is challenging.
Human and Wildlife Health Implications of STXS and DA
During toxic HAB events, STXs and DA accumulate in filter-feeding marine organisms such as zooplankton, clams, worms, and fish. These accumulated toxins can be passed to upper trophic levels, where they can cause severe illness and death of humans and wildlife (Figure 1; Landsberg et al., 2014). Although both STXs and DA are detected throughout food webs in many parts of the world, their known impacts vary by taxa and geographic region. Globally, STXs have been responsible for mortality in fish, invertebrates, sea turtles, seabirds, and marine mammals (Landsberg et al., 2014). In contrast, reports of DA-associated wildlife mortality have been limited to marine mammals and seabirds, mostly along the west coast of the contiguous United States (Landsberg et al., 2014). Both STX- and DA-producing HABs are present in Alaskan Arctic ecosystems and have the potential to impact a wide variety of wildlife species, as well as humans. Although no confirmed cases of STXs- or DA-associated poisonings have yet been reported in wildlife or humans in the Alaskan Arctic, these toxins have been documented in zooplankton, clams, worms, planktivorous fish, marine mammals, and seabirds, providing evidence of exposure to HAB toxins across multiple trophic levels (Lefebvre et al., 2016; Van Hemert et al., 2021a; Lefebvre et al., 2022; Figure 5).
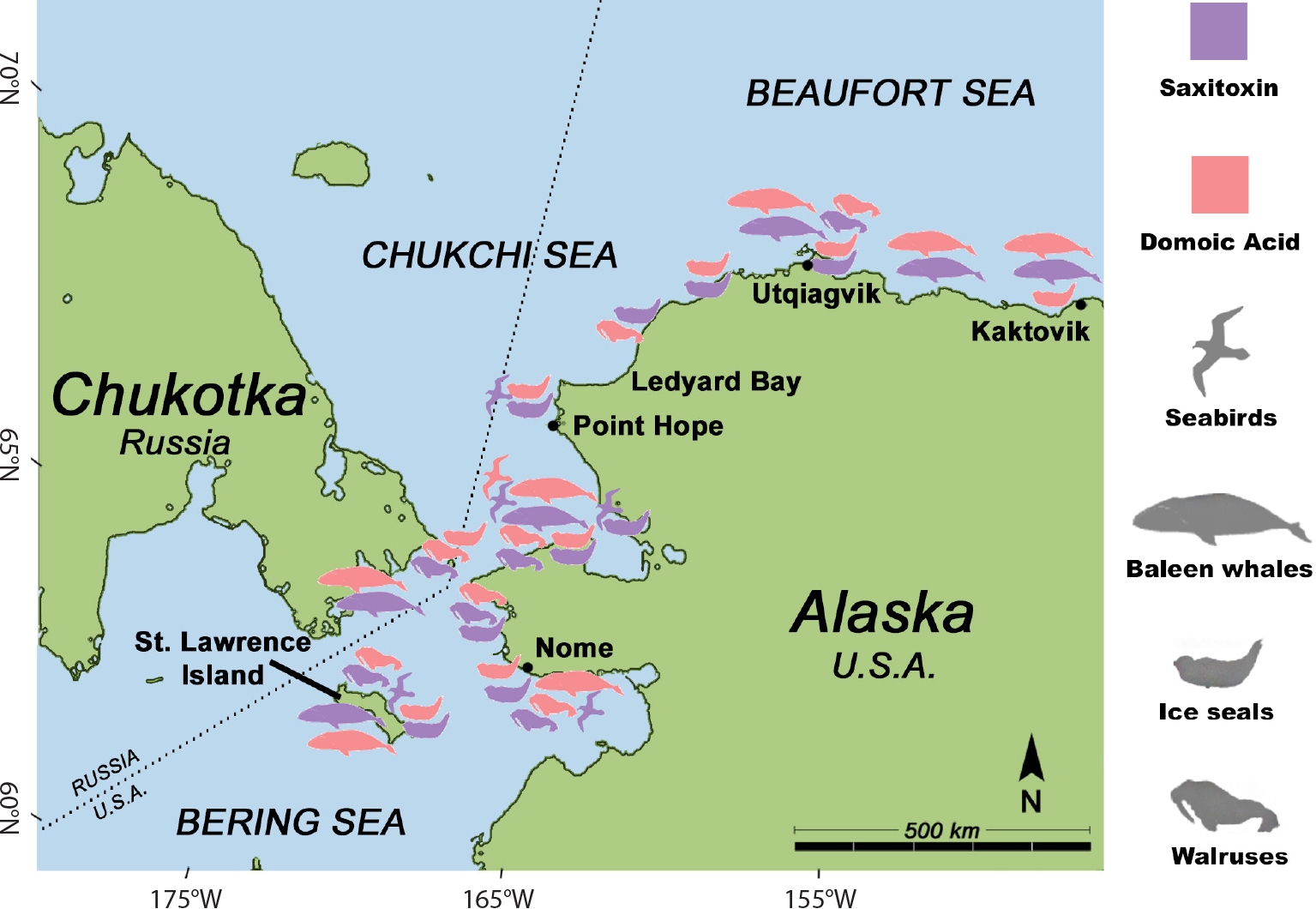
FIGURE 5. Map of locations where stranded or subsistence harvested marine mammals and seabirds have tested positive for saxitoxin (STX, purple) and/or domoic acid (DA, red) in samples collected from St. Lawrence Island to the Beaufort Sea from 2001 to 2021. Taxa include seabirds, baleen whales, ice seals, and walrus. Algal toxin detection limits for wildlife samples were approximately 4 ng DA/g for domoic acid and 3 ng STX eq./g for saxitoxin (Lefebvre et al., 2016). > High res figure
|
Typically, STXs and DA are found at highest concentrations in gastrointestinal tracts, livers, and kidneys of marine organisms, a pattern that has also been observed among Arctic seabirds and marine mammals (Lefebvre et al., 2016; Van Hemert et al. 2021a). Planktivorous fish have also been shown to depurate toxins quickly and have the highest concentrations (>90%) in viscera (Lefebvre et al., 2001). However, some species of clams, like the razor clam (Siliqua patula), have been shown to contain DA in edible tissues at levels above regulatory limits and to remain toxic for over a year (Wekell 1994). More species-specific information on uptake and depuration is needed to determine which tissues could harbor potentially harmful levels of toxins and whether human risk reduction is possible through specific harvesting or food preparation measures.
Based on available food web data from the Alaskan Arctic, STXs appear to be a more urgent threat to wildlife and human health than DA. Across multiple taxa, STXs have been detected more frequently and at higher relative concentrations than DA (Lefebvre et al., 2016; Van Hemert et al., 2021a). DA levels measured in zooplankton, clams, worms, fish, and marine mammals from the Chukchi Sea and Alaskan Beaufort Sea regions were well below the seafood safety regulatory limit (20 µg DA/g tissue; author Lefebvre, unpublished data). In contrast, concentrations of STXs at or near the seafood safety regulatory limit (80 µg STX eq./100 g tissue) were measured in Alaskan Arctic clams, zooplankton, and Pacific walruses (Lefebvre et al., 2022).
The higher levels of STXs found in clams make them the most toxic vectors identified in the Alaskan Arctic region to date, suggesting that they present a distinct risk to marine mammals and humans. This finding agrees with previous studies showing higher prevalence and concentrations of STXs in clam-feeding walruses and bearded seals compared to other marine wildlife that feed on fish or zooplankton, such as spotted seals, bowhead whales, and seabirds (Lefebvre et al., 2016; Hendrix et al., 2021; Van Hemert et al., 2021a; Lefebvre et al., 2022).
In addition to known shellfish vectors, other potential sources of STXs and DA in Arctic food webs warrant consideration. Forage fish are known to accumulate STXs in regions where A. catenella blooms are common, including the Gulf of Alaska (Van Hemert, 2021b). In a 2019 survey of Arctic forage fish, STXs were detected at low to moderate concentrations in fish collected from the northern Bering Sea and Bering Strait region, but not in fish collected from the northern Chukchi and Alaskan Beaufort Seas (Lefebvre et al., 2022). More samples are clearly needed to determine the role of fish as potential vectors in the Alaskan Arctic.
Nontraditional vectors (Deeds et al., 2008) include zooplankton and other marine invertebrates that are important food sources for many wildlife species in the Arctic. The high energetic demands of northern seabirds, whales, and other cold-adapted taxa may result in the consumption of harmful quantities of toxin during HAB events, even when prey toxin concentrations are relatively low (Van Hemert, 2021b). It is also important to note that toxic doses and susceptibility among marine mammals and seabirds have not yet been determined, and impacts on wildlife health cannot be inferred from human seafood safety guidelines (Lefebvre et al., 2016; Van Hemert et al., 2021a). As knowledge of HABs in the Alaskan Arctic expands, additional sampling as well as targeted experimental studies are needed to determine species’ sensitivity to better understand risks to piscivorous seabirds and other marine wildlife.
Although many questions remain about the ecosystem-level impacts of HABs in the Alaskan Arctic, growing evidence indicates the possibility of an emerging wildlife health issue. Recent reports demonstrated increasing prevalence of DA in marine mammals (Hendrix et al., 2021), along with possibly harmful concentrations of STXs in seabirds associated with known mortality events (Van Hemert et al., 2021a). These findings, combined with projections of more frequent and intense STX-producing HABs due to warming ocean conditions in the Arctic (Anderson et al., 2021a), suggest that marine wildlife (and the people who harvest and consume them) may face growing exposure risks.
The potential impacts of HABs on the food web of the Alaskan Arctic are far-reaching, as marine wildlife of the northern Bering, Chukchi, and Beaufort Seas are essential to the nutritional, cultural, and economic well-being of coastal communities. HAB toxins present two major hazards to coastal communities: (1) illness or mortality via direct consumption of potentially toxic seafoods, and (2) compromised food security via both avoidance of foods due to fear of toxicity and the loss (through mortality) of essential marine resources used for food. Of note are recent studies demonstrating that repetitive, low-level exposure, especially in subsistence populations and high fish/shellfish consumers, can have negative outcomes (e.g., problems with everyday memory; e.g., Grattan et al., 2018).
It is essential to recognize not only the acute toxicity risks posed by HABs but also the multifaceted impacts on traditional food sources and culture. The low-level presence of STXs and DA is not new to northern and western Alaskan waters (Lefebvre et al., 2016), but local concerns now reflect both a rapid increase in knowledge about HABs as well as the associated shift in perceptions of food safety and availability.
Challenges and Approaches to Monitoring and Management
The Alaskan Arctic faces multiple challenges in monitoring and responding to HABs, some of which are unique to the region. A detailed analysis is beyond the scope of this review; here, we summarize the main challenges and suggest possible approaches.
Efforts to monitor and manage HABs in the region are hindered by a lack of information, limited infrastructure, and unique spatial challenges inherent in Alaskan land- and seascapes (Figure 6). Foremost among the challenges is the need to provide coverage across large stretches of sparsely populated coastline. Transportation and communication infrastructure is limited and often impacted by harsh weather. As a first step toward enhanced communication, the Alaska Harmful Algal Bloom Network (AHAB: https://aoos.org/alaska-hab-network/) has been established to share information among a diverse group of scientists and interested stakeholders throughout Alaska (Anderson et al., 2019). This stakeholder-initiated effort is currently funded by federal appropriations that are subject to funding uncertainties, and thus a more stable state-supported communications strategy and network might be needed to enhance and sustain HAB response.
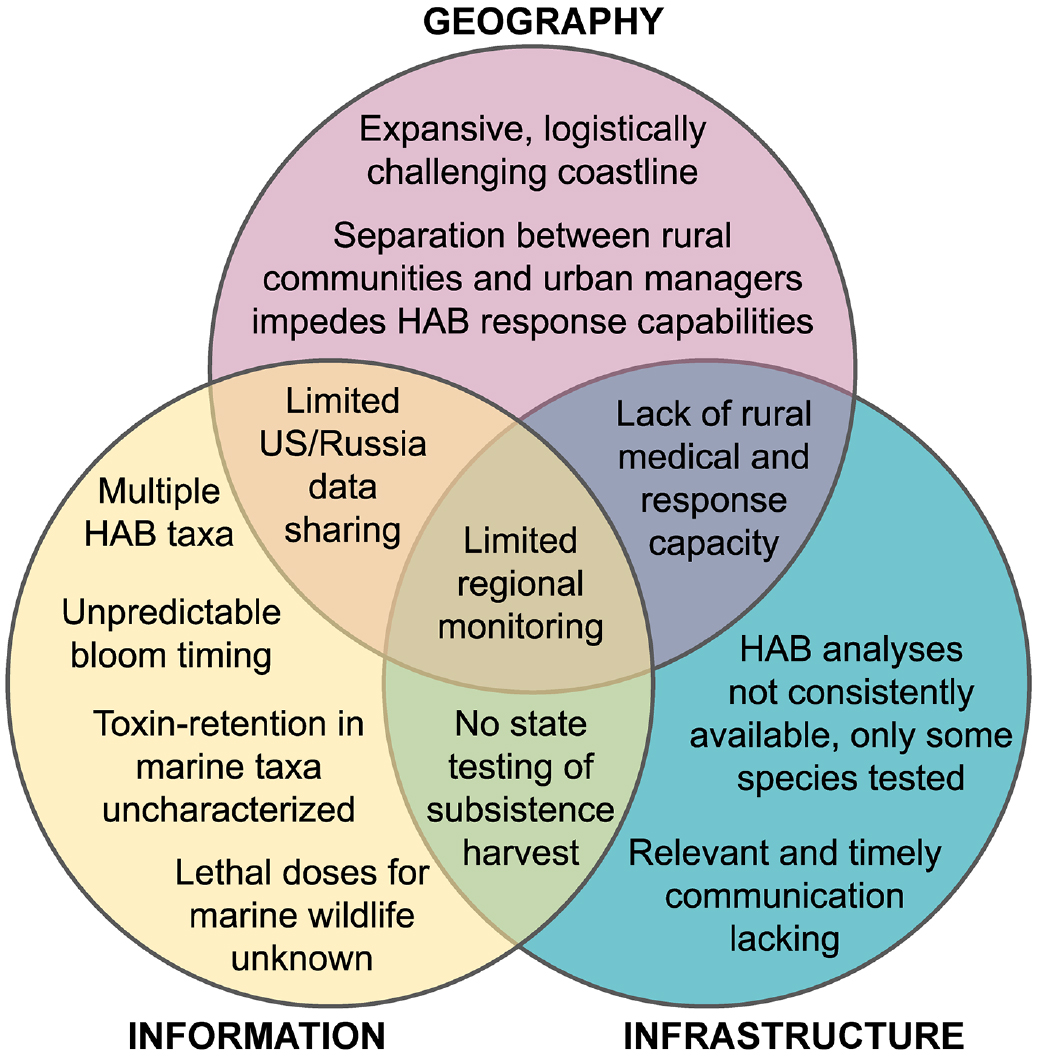
FIGURE 6. Overview of challenges to HAB monitoring and management in the Alaskan Arctic. Challenges derive from lack of complete information, limitation of available infrastructure, and spatial logistics unique to Alaskan geography. > High res figure
|
Scientists, managers, and agencies concerned with HAB events are primarily urban-based in Alaska, far from the northern and western coasts, so they are largely reliant on coastal communities for awareness of a HAB event or human medical emergency. The lack of a robust infrastructure contributes to a high-risk situation, as recently demonstrated in 2020 with the first human HAB/PSP fatality since 2010 in Alaska, and the first reported fatality in western Alaska (Alaska DHSS, 2020).
An additional complication is that resource managers, community leaders, and regulatory officials must deal with multiple HAB toxins and algal species that occur in different seasons and locations, with blooms that are highly episodic and as yet unpredictable. HAB toxins can also accumulate in, and affect, a diverse suite of marine species that are food sources for local communities. The State of Alaska tests all commercial shellfish harvested, but there is no state-run testing program for the recreational and subsistence harvest. With no federally authorized commercial harvest of seafood in the Chukchi and Beaufort Seas, all seafood is harvested on a noncommercial basis and thus is not included in state-funded HAB monitoring.
Given the geographic and logistical constraints of monitoring HABs in the Alaskan Arctic and the lack of a state-funded toxin testing program for noncommercial harvest, the marine ecosystems of the Alaskan Arctic, and the people who rely on them, are at risk. A monitoring approach to be considered would be the establishment of a local or regional monitoring program, perhaps modeled after the program run by the Sitka Tribe of Alaska. This effort is focused on the Gulf of Alaska and is limited to shellfish, but staff and facilities for HAB toxin analysis are in place to serve community concerns about HABs through shellfish toxin testing, paid for by the users. Currently, there is no community-based HAB testing program in the Alaskan Arctic, and if one is established, it is important to recognize that shellfish are only a minor and occasional component of diets in the region. Regional monitoring programs will thus need to develop protocols and capabilities to test seabirds, fish, and marine mammals as well. Ongoing research by university, agency, and other partners can provide information about the presence of HAB toxins in fish and wildlife, but current sampling efforts are limited, and many diagnostic tools used are not directly applicable to food safety assessments.
Experiences in other regions of the world suggest that a plankton screening program to detect HAB cells in coastal waters could also be a useful element in local or regional monitoring programs. Local monitoring using plankton nets and inexpensive microscopes is common in many areas subject to HABs (Trainer et al., 2014), and training and funding to establish this capability should be a high priority activity in the Alaskan Arctic going forward. Given the many existing and growing challenges to coastal communities, however, citizen or volunteer plankton monitoring programs may not be feasible in this region. The direct testing of seafood harvest should therefore be considered, though the manner in which this could be accomplished is unclear given limited transportation infrastructure and analytical capabilities.
With respect to ecosystem health and food security, potential impacts from STXs and DA to most marine wildlife in the Alaskan Arctic are unknown and thus there is no firm guidance to provide for the safety of coastal communities. Ongoing grant-funded research programs will soon provide data of this type, and it will be critical to include effective communication and outreach plans to provide coastal communities the data and implications as they become available.
Yet another concern is that the marine ecosystems of the Alaskan Arctic are shared with the Russian Federation, and transboundary communications can be logistically, politically, and bureaucratically challenging. Efforts are needed to promote collaborations in research, monitoring, and communications to protect shared wildlife resources and public health.
Recent technological advances in HAB monitoring may also provide important monitoring tools for the region. Given frequent cloud cover and the lack of HABs of sufficient density to be visible from space, traditional satellite remote sensing has limited utility in the Arctic. Of more value are new sensors capable of detecting and quantifying HAB cells and toxins in situ (Doucette and Kudela, 2017). A promising development in this regard is the advent of ocean observing systems—arrays of moored and mobile instruments that can collect and transmit data continuously from remote locations to shore-based scientists and managers. Instruments capable of measuring HAB cells and/or toxins already exist, such as the Imaging Flow Cytobot (IFCB), a high-speed, submersible microscope that can autonomously operate 24/7 and take hundreds of thousands of images of phytoplankton daily (Olson and Sosik, 2007). Machine-learning algorithms then identify and enumerate algal species such as the major HAB taxa described here, providing near-real-time data on HAB threats (Brosnahan et al., 2015). These instruments can be deployed on docks or piers or placed on fishing or research vessels for analysis of underway samples (Figure 7). It should also soon be possible to deploy them seasonally on autonomous surface vehicles (ASVs) equipped with solar power and communications hardware. Given the demonstrated northward transport of Alexandrium blooms through the Bering Strait and into the Chukchi Sea, an IFCB-equipped ASV located near Kotzebue Sound could provide valuable data on incoming HABs, for example.
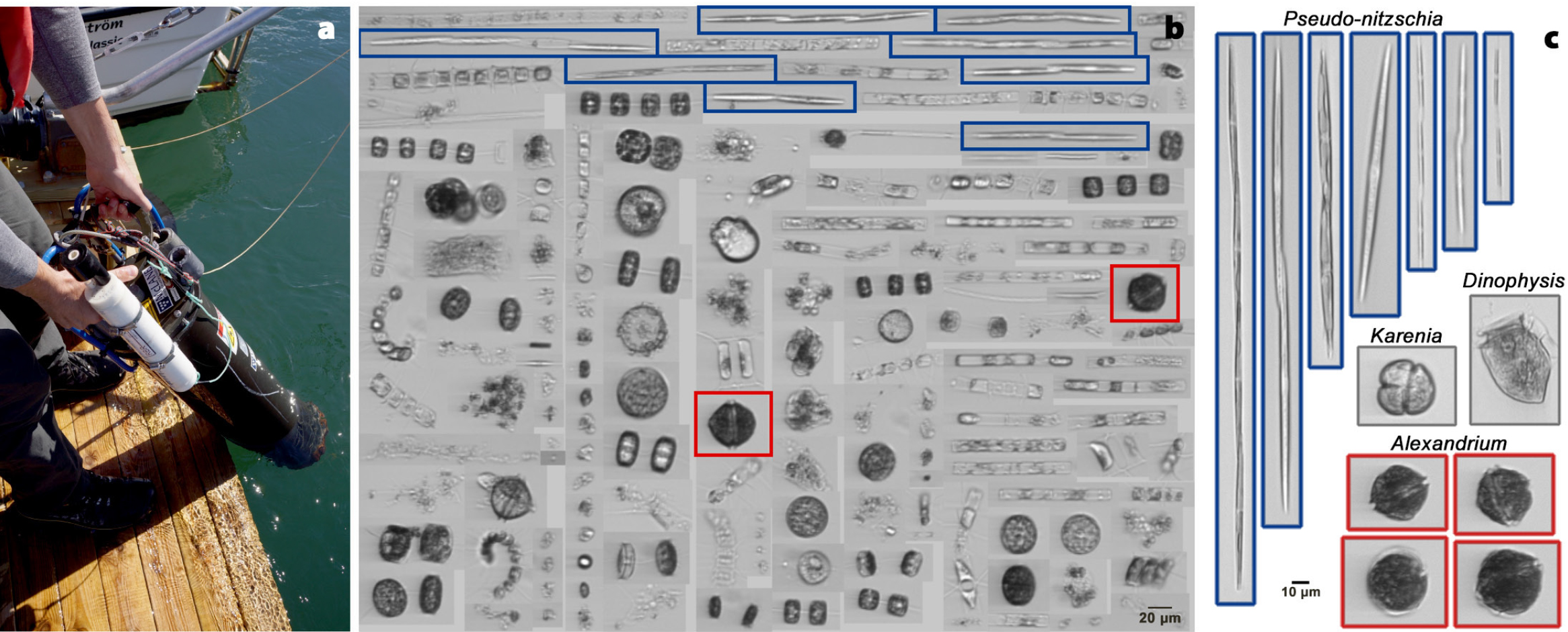
FIGURE 7. (a) An Imaging FlowCytobot (IFCB). Image credit: Michael Brosnahan, WHOI (b) Examples of phytoplankton imaging data that the instrument produces. A single water sample collected from shipboard underway seawater in the Chukchi Sea during an August 2018 cruise contained Alexandrium (red boxes) and Pseudo-nitzschia (blue boxes). (c) Post-processing of IFCB imagery classifies cells by type, allowing easy identification and quantification of HAB cells, including Alexandrium, Pseudo-nitzschia, Karenia, and Dinophysis. > High res figure
|
These are some of the approaches that could be taken to begin to monitor for and respond to HAB events in the Alaskan Arctic. Many other regions of the world face recurrent HABs that contaminate seafood products and affect ecosystem health, yet it has proven possible to protect human health and sustain fisheries and other ecosystem services through informed management actions. The unique nature of the Alaskan Arctic, the lack of scientific understanding of HAB impacts on marine wildlife, and the reliance of coastal populations on noncommercial harvesting for nutritional, cultural, and economic well-being poses new and significant challenges that need to be immediately addressed as this region continues to warm and the potential impacts from HABs expand.
Acknowledgments
The authors acknowledge that the Alaskan Arctic as described here includes the lands and waters of the Inupiaq, Saint Lawrence Island Yupik, and Central Yupik peoples. Funding for DMA, RSP, EF, PL, and MLR was provided by grants from NSF Office of Polar Programs (OPP-1823002 and OPP-1733564) and NOAA’s Arctic Research program (through the Cooperative Institute for the North Atlantic Region [CINAR]; NA14OAR4320158 and NA19OAR4320074), and for DMA, KH, and KAL through NOAA’s Center for Coastal and Ocean Studies ECOHAB Program (NA20NOS4780195). Additional support was provided for DMA, MLR, and EF by the US National Park Service Shared Beringian Heritage Program (P21AC12214-00). We also thank Natalie Renier (WHOI Graphic Services) and Emily Bowers (Northwest Fisheries Science Center) for creating figures. Any use of trade, firm, or product names is for descriptive purposes only and does not imply endorsement by the US Government. This is ECOHAB Contribution number 1007.