Introduction
The element thorium is very insoluble in the ocean, a property shared by many other trace elements (Goldberg, 1954). This characteristic makes thorium useful as a tracer (Krishnaswami et al., 1976). The upper continental crust is already low in thorium—there are about 10 micrograms of thorium per gram of crust (Rudnick and Gao, 2014). In seawater, its abundance is even smaller—the average dissolved thorium concentration in the ocean is ~0.1 picomolar, or 0.4 femtograms (0.4 × 10–15 g) Th per gram of seawater (Schlitzer et al., 2018). In other words, thorium is 25 billion times more concentrated in the crust compared to seawater. The little thorium that does make it into the dissolved phase in seawater (operationally defined as that which passes through a filter of ~0.45 µm) comes from partial dissolution of crustal material or from the radioactive decay of a soluble parent isotope. Because of its dislike of an aqueous environment (Santschi et al., 2006), dissolved thorium in the ocean will be adsorbed onto particulate material, or “scavenged,” and eventually become buried in marine sediments.
The insolubility of thorium, and its well-known production from the decay of its parent isotope, are the key ingredients for making thorium a stopwatch in the ocean. Radioactive decay chains, if left undisturbed, tend toward secular equilibrium. This means that the radioactivity (in decays per second) of parent and daughter isotopes is equal. This radioactivity balance cannot always exist in seawater because as soon as thorium is injected into water, it quickly becomes attached to sinking particles and is removed from the water. On timescales of weeks to months, we can use the extent of removal of thorium compared to its parent to derive a timescale of removal. On longer timescales of years to decades, the extent of thorium removal from the dissolved phase is virtually complete. In the longer-term case, we can usually assume that all of the thorium that was introduced into the water over a given period of time will have been removed and is either traveling downward in the water column on sinking particles, or is completely removed from the water column and buried in the sediments.
Tracing Particle Export with 234Th
We begin this isotopic perspective with the shortest-lived, yet heaviest by weight, of the isotopes considered here, 234Th. It is the radioactive progeny of the most abundant form of uranium in seawater, 238U, which is well-mixed throughout the ocean (Figure 1). 234Th itself has a half-life of 24 days. The shorter an isotope’s half-life, the faster that secular equilibrium with its parent is approached. The scavenging removal process needs to happen fast enough to occur before the ongoing decay of 238U can resupply the 234Th (Bhat et al., 1969). Deficits of seawater 234Th activity compared to its production by 238U decay typically occur most conspicuously in the upper 150 m of the water column (Buesseler et al., 1992; Ceballos-Romero et al., 2022), where the largest vertical sinking, or export, of particulate matter occurs, as well as near the seafloor, where resuspension of sediments results in enhanced scavenging (Bacon and Rutgers van der Loeff, 1989).
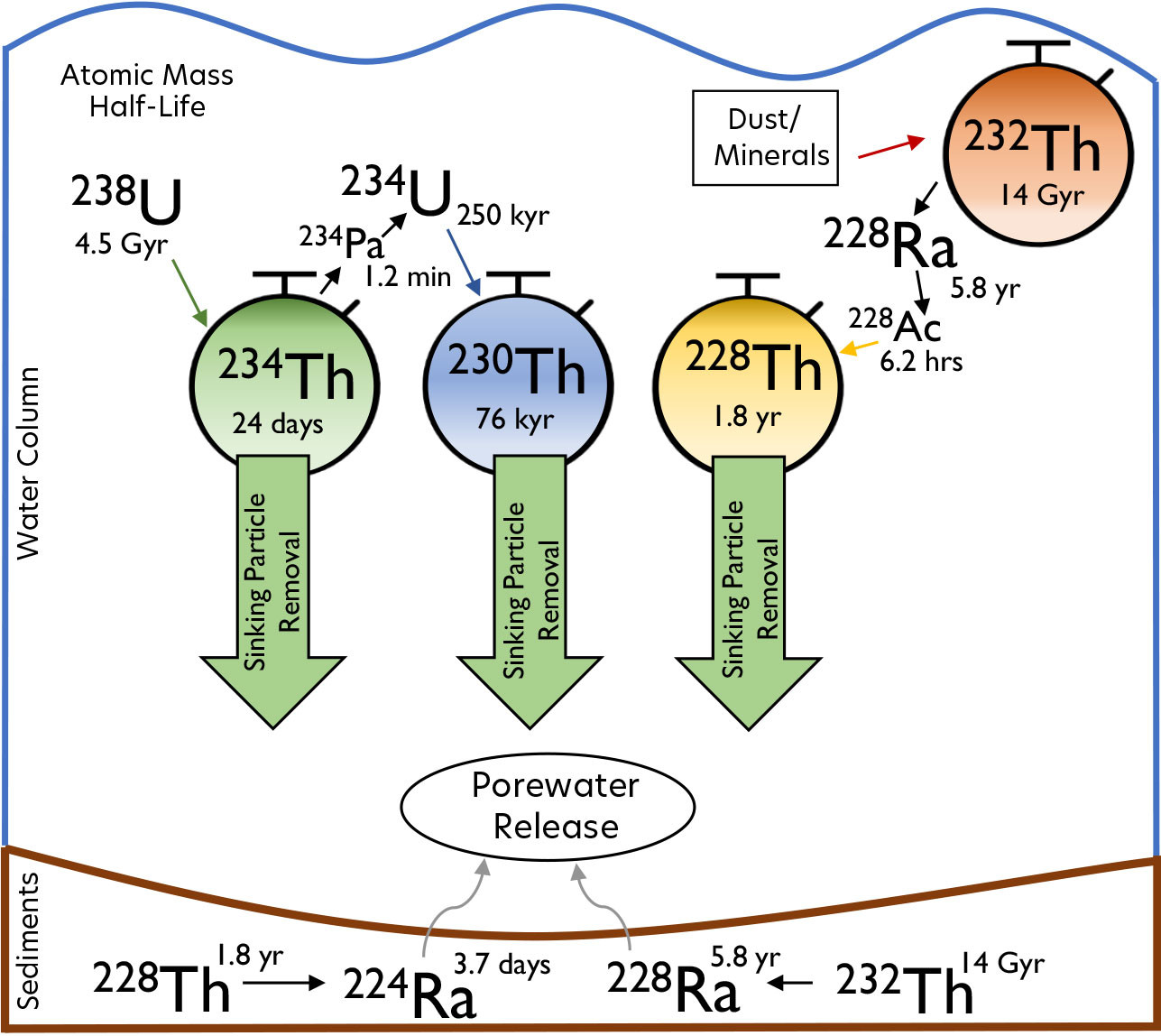
FIGURE 1. Thorium isotopes as stopwatches used for oceanographic applications. 232Th is a primordial isotope with its own decay chain, including 228Ra, 228Th, and 224Ra. 234Th and 230Th are radiogenic and are part of the 238U decay chain. Many other trace metals are involved in the processes of sinking particulates and porewater release, for which thorium provides important timescale information. The intermediaries 234Pa and 228Ac are essentially ignored in these processes because they are very short-lived. Note that the decay chains extend beyond the isotopes shown here (Geibert, 2008; Rutgers van der Loeff and Geibert, 2008), ultimately into stable isotopes of lead, but these are omitted for clarity. > High res figure
|
A specific elemental removal rate is difficult to otherwise estimate in the environment and is very useful in considering an element’s turnover, or residence, time. In an ecosystem, the residence time of the micronutrient iron, for instance, is a critical timescale for all the living things that require this iron for their biological functioning. Working with data from the international GEOTRACES program, Black et al. (2020) quantified the removal rate of 234Th from the upper ocean throughout the North Atlantic and South Pacific using the deficit method described above. Figure 2 shows some of the North Atlantic 234Th data (Owens et al., 2015), which exhibits a characteristic “deficit” of 234Th in the upper ~150 m, the extent of which is used to estimate particle export flux from the upper water column. Black et al. (2020) make the connection to trace element cycling by taking the removal rate analysis one step further and predicting how much iron is being removed by this particle export process. These authors further constrain upper ocean iron residence times on a vast scale by comparing 234Th-based Fe removal rates with the measured inventory of Fe. The majority (67%) of new estimates of the total Fe residence time in the upper 250 m of the ocean fall within 10–100 days, a shorter timescale than previously thought, in some cases an order of magnitude shorter. Note that the 234Th stopwatch is well positioned to track this timescale of weeks to months as it approaches 5 times 24 days, or the timescale on which 234Th would return to secular equilibrium.
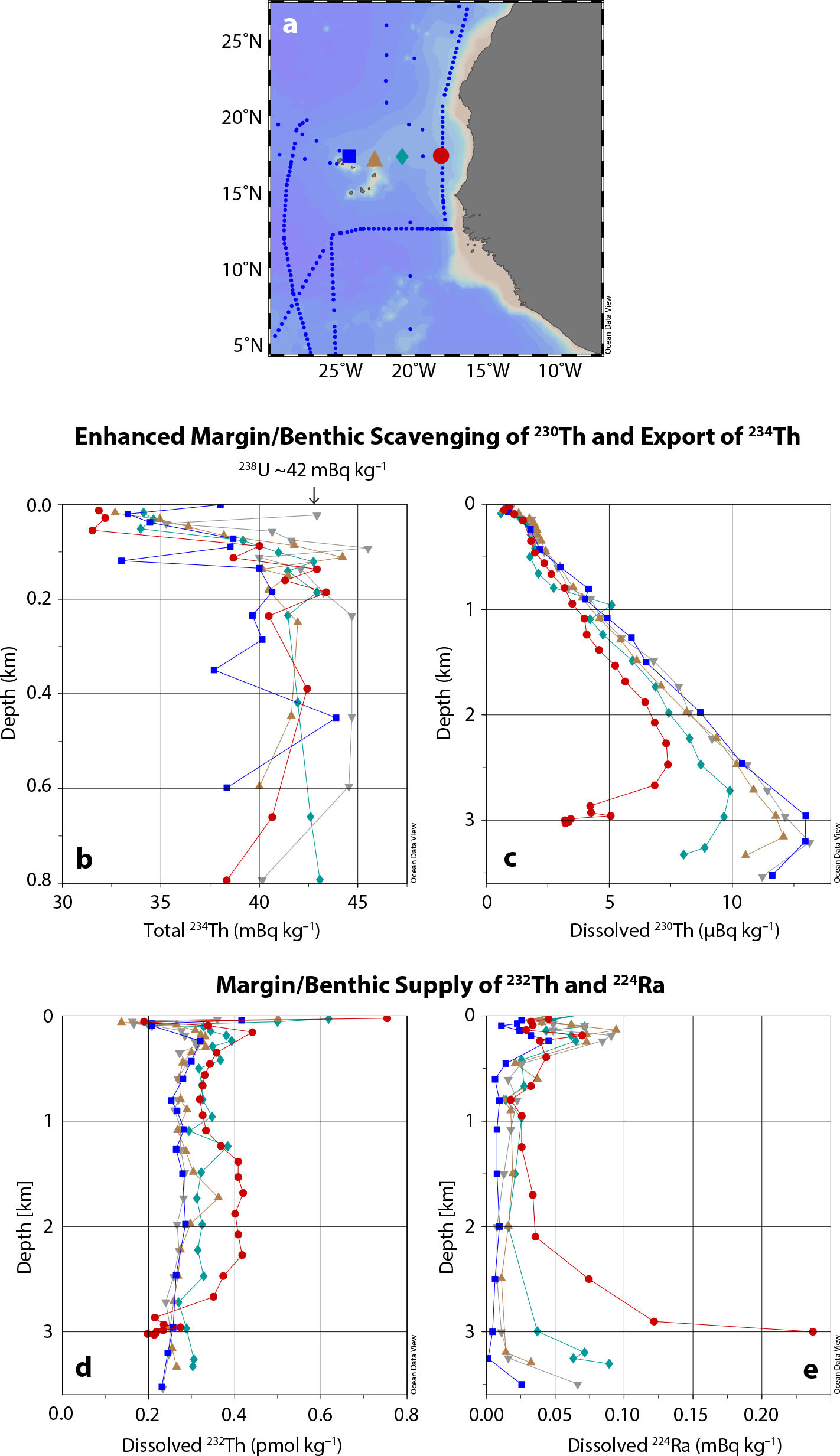
FIGURE 2. GEOTRACES data demonstrating variations in Th isotope behavior from North Atlantic section GA03. (a) On the map of the West African margin, plotted station data are shown as colored symbols, and small blue dots indicate other available data in the GEOTRACES Intermediate Data Product. The depth profiles show (b) total (dissolved + particulate) 234Th (Owens et al., 2015) and also the uniform activity of 238U (note unique depth scale), (c) dissolved 230Th (Hayes et al., 2015), (d) dissolved 232Th (Hayes et al., 2018b), and (e) dissolved 224Ra (decay product of 228Th; Charette et al., 2015). > High res figure
|
Tracing Benthic Element Supply with 228Th (and Ra)
Moving up in half-life, 228Th offers another kind of stopwatch, with applications for both trace element removal and trace element supply from benthic sediments. This isotope is sandwiched in a decay chain between a series of radium isotopes. The parent 228Ra (half-life 5.8 years) undergoes two beta decays and a short-lived intermediary to form 228Th (half-life 1.9 years), which subsequently alpha decays into 224Ra (half-life 3.6 days). In contrast to all Th isotopes, Ra is relatively soluble in seawater. While 228Th :228Ra considerations can be used to quantify particulate scavenging removal (Figure 1; Hayes et al., 2018a) similar to 234Th, we focus here on a different application, using 228Th as the parent isotope rather the progeny.
The 228Th in sediments supplies a constant source of 224Ra to sediment porewaters (Figure 1). Some of that 224Ra escapes into bottom seawater through processes of sediment irrigation and advective flows, and this 224Ra source is demonstrated in the North Atlantic dataset shown in Figure 2 (Charette et al., 2015). The deficit of 224Ra within sediment porewaters with respect to its parent 228Th can further be used to derive a flux of 224Ra to bottom seawater (Cai et al., 2014). Extrapolation to benthic porewater fluxes of other elements can be accomplished using the porewater concentration gradients of those other elements. Shi et al. (2019) used this 224Ra/228Th method to study coastal sediments throughout eastern China to estimate benthic fluxes of iron from the sediments. Although iron is very insoluble in oxygenated seawater, in the conditions found in sedimentary porewaters, Fe can be released into the dissolved phase (likely as colloids) in great proportions through both reductive and non-reductive pathways to build up concentrations much larger than that found in seawater (Homoky et al., 2021). The Shi et al. (2019) study investigated relatively shallow sites, with water depths ranging from 0 to 80 m. Within that range, they found much greater benthic iron fluxes at sites shallower than 20 m depth, a trend the authors ascribe to greater mixing and wave energy irrigating the porewaters at the shallower sites. They also found that the Fe fluxes derived from the Th method were up to orders of magnitude larger than those detected with previous methods using benthic lander chambers that can make very detailed porewater measurements at a particular landing site. It may be that the Th method is a better spatial integrator of fluxes spread out over a particular coastal area, and the benthic lander method presents a sampling bias. Much more is to be learned about benthic pore water fluxes as this method is applied to other regions. In particular, benthic fluxes of copper and aluminum have long been thought important to the overall cycling of these elements, which could also be quantified in this way. A similar method can be applied to the longer-lived 228Ra, whose parent is 232Th (Figure 1; e.g., Sanial et al., 2018), with some slightly different assumptions (Charette et al., 2016).
Tracing Scavenging and Particle Fluxes with 230Th
There is a large jump in time to the next longest-lived Th isotope, 230Th, with its half-life of 76,000 years, but it can provide information on particle sinking rates on longer timescales. Similar to 234Th, 230Th is produced in the ocean by another uniformly distributed uranium isotope, 234U (Figure 1). Once injected into the dissolved phase by alpha decay, 230Th does not stay in seawater for 76,000 years, because it is so insoluble that it is scavenged and buried in the sediments on the timescale of years to decades (Henderson and Anderson, 2003). Accordingly, within the water column, 230Th can record events on the timescale of its water column residence rather than on the timescale of its radioactive half-life. In the sediment, 230Th inventories can record changes in particulate matter burial up to ~400,000 years ago (Costa et al., 2020). We won’t go that far back in time here, but past ocean particle fluxes are important, for example, for understanding the ocean’s uptake of carbon dioxide and global climate.
As in the case of 234Th-derived particle fluxes of Fe, 230Th can also be used to estimate the downward sinking flux of other particulate elements. Dissolved 230Th in the water column is generally inversely proportional to the intensity of particle scavenging (Figure 2). However, for the particulate phase 230Th, the assumption would be that all of the 230Th produced by 234U decay above a given depth horizon in the water column is settling toward the seafloor. This known flux can be used as a normalization to estimate the sinking flux of other elements (Bacon and Rosholt, 1982). Especially deeper in the water column (~2 km), 230Th-normalized flux on water column particles is one of the only methods for estimating a sinking particulate flux, aside from the traditional technique of deep-moored sediment traps.
Rahman et al. (2022) used the particulate 230Th technique to investigate the ocean cycle of particulate barium in GEOTRACES transects. While barium is not an essential micronutrient like some other trace metals, it may be a useful proxy for biological productivity. Barium tends to precipitate as barite (or barium sulfate) in sinking aggregates of particulate organic matter, but it is relatively well preserved as it sinks in the water column and is subsequently buried, whereas almost all of the organic matter tends to be respired before burial. By comparing sinking rates of barium in the deep water column with barium burial rates in the sediments, these authors were able to determine that preservation efficiencies of this potential proxy for organic matter export varied a great deal (between 17% and 100% across the North Atlantic and South Pacific). This study raises some important questions on the reliability of particulate barium as a proxy in the sediments and warrants further investigation into the controls on barite preservation, part of which may involve the seawater barite saturation state (Mete et al., 2023).
Tracing Dust and Benthic Supplies with 232Th
The isotope 232Th makes up nearly all (99.98%) of the thorium in Earth’s crust on a per weight basis and can provide critical information on trace element supply from mineral sources. Mineral dissolution is one of the main suppliers of the micronutrient iron to the ocean, and its scarcity can limit biological rates in certain regions. Iron measurements alone cannot always determine its main sources, and therefore tracers of its supply are quite useful to oceanographers.
232Th does not come from the decay of anything but is primordial in the sense that this element was included in the star dust that coalesced to originally form our planet. Its extremely long half-life (14 billion years) means that on most timescales considered in the water column or sediments, the effect of its own radioactive decay on its concentrations can be ignored. Therefore, we don’t truly use 232Th as a stopwatch itself, but we can use the timescale information from other thorium isotopes to measure how quickly 232Th is moving around. While potentially any of the other stopwatch isotopes could be used for this, 230Th has been paired most often, because both of these isotopes are abundant enough to be routinely measured by mass spectrometry (i.e., counting the number of atoms in a sample). The shorter-lived 234Th and 228Th are still measured by counting their radioactive decay and are truly miniscule on a per atom basis.
It is worth mentioning briefly here what advances the community has made in analyzing thorium isotopes in the environment, especially for the long-lived isotopes that have the lowest activities. Some of the first precise measurements of 232Th and 230Th reported in seawater (Moore and Sackett, 1964) were made using ~200 liter water samples, which was roughly the necessary size sample needed to detect these isotopes using alpha decay counting. With advances in mass spectrometry in the 1980s and 1990s, the first paired mass spectrometry measurements of 232Th and 230Th indicated that sample volume could be reduced to 1 liter (Roy-Barman et al., 1996). This reduction in volume needed drastically increased the sample throughput that could be achieved and ultimately made possible GEOTRACES section-type data for these isotopes (e.g., Figure 2).
As mentioned at the beginning, the main source of the dissolved phase of 232Th in the ocean is the partial dissolution of crustal material. In the remote open ocean, the primary source of crustal material is wind-blown dust from upwind desert regions. The North Atlantic dataset in Figure 2 shows that increased 232Th concentrations near the West African margin are likely due to both dust and margin sediments. Thinking about iron sources rather than iron removal, dissolved 232Th input rates, estimated by measuring 232Th and 230Th together, can be converted into input estimates of dust to the ocean (Hsieh et al., 2011) and of the iron coming out of that dust. Pavia et al. (2020) recently did this in a GEOTRACES process study in the biological “desert” of the subtropical South Pacific. Dust input to this region of the ocean is one of the lowest in the world, so low in fact that Pavia et al. determined that there must be efficient recycling of the dust-derived Fe here in order to support the prevalent levels of the Fe-requisite enzymatic function of nitrogen fixation found in cyanobacteria.
Thorium-based dust estimates still face uncertainties, including assumptions about how much Th dissolves from dust, where exactly in the water column it dissolves, and how exactly to apply Th residence times based on 230Th. An intercomparison of several methods for estimating dust deposition in the North Atlantic, including aerosols, 7Be, sediment traps, and thorium, reveal agreement between the methods within about a factor of 5 (Anderson et al., 2016), demonstrating a non-trivial uncertainty that will improve with further development of these methods.
Advances and Future Opportunities
To summarize, we have learned that the thorium stopwatches are useful for quantifying trace element supply and removal terms in the ocean’s biogeochemical cycle. Thorium-based estimates have refined iron residence times and iron supply rates from both dust deposition and benthic sediment release. While the role of iron as a limiting nutrient to ocean ecosystems has garnered that element much attention, the thorium isotopes are also helping to constrain the movement of other trace metals, including Cu, Al, and the paleo-proxy Ba as mentioned, as well as other micronutrient metals Co, Cd, and Mn (Black et al., 2019) and the pollutant Pb (Lanning et al., 2023), not covered here.
There is still more to discover about thorium isotope behavior in the ocean, however. The nature of the dust dissolution processes, thorium scavenging itself, and the assumptions behind the paired use of 232Th and 230Th are all still relatively unexplained in terms of mechanistic detail. For instance, 230Th-based 232Th fluxes tend to increase with depth in the upper water column, which is not realistic given a source from dust dissolution in the surface ocean. Dissolved 232Th fluxes can also represent metal supply from margin sediments (Pérez-Tribouillier et al., 2020) in addition to, or in the absence of, dust sources, sometimes creating a disentanglement issue for these sources (Hayes et al., 2022). Ocean biogeochemical modeling of the thorium isotopes is ongoing and has potential to help clarify both removal rate and supply rate issues since these models can now be constrained by the growing GEOTRACES database. Additionally, while it is often assumed that Th adsorbs onto any particulate matter agnostically, marine particle types have systematic variation in size partitioning (Subhas et al., 2023). This size partitioning affects specific surface area for adsorption, as well as the chemical adsorptive capacity of particle types such as organic matter functional groups. Fundamental surface chemistry modeling and measurements may be helpful in shedding light on these processes that, up until now, have mainly only been investigated through their impacts on radioactive disequilibria.
Nonetheless, the results so far for Th-based fluxes and timescales are clearly pushing toward refining these useful approaches. Next time you have a geochemical question on trace element supply or removal at sea, consider using one of the thorium stopwatches!
Acknowledgments
This work was in part funded by US NSF award 2048863. The international GEOTRACES program is possible in part thanks to the support from the US National Science Foundation (Grant OCE-2140395) to the Scientific Committee on Oceanic Research (SCOR).