The words “microplastic,” “single-use plastic,” and “microfiber” have become commonplace in newspaper headlines, radio programming, and policy statements. Stories about dead whales washing ashore with bellies full of plastic bags and images of turtles with plastic straws up their noses can easily be found online. At local elementary schools, parents are asked to pack zero-plastic-waste lunches for their children, and global conferences focused on the world’s most pressing environmental issues all include plastic debris along with climate change and fisheries exploitation. Fourteen years ago, when I was applying for graduate school, the words plastic and pollution were not yet united to define the growing environmental issue we all know today as “plastic pollution.” Today, the United Nations is considering a new international agreement focused on plastics in the environment.
The Story Begins in the Open Ocean
The history of research around plastic pollution begins in the middle of the ocean basins, thousands of kilometers from land, in the central gyres (Figure 1a). The first scientific findings of marine plastic debris were published in the journal Science in 1972, reporting on small plastic particles found in the Sargasso Sea (Carpenter et al., 1972; Carpenter and Smith, 1972). More than a decade later, in 1986, undergraduates aboard a tall ship began counting pieces of small plastic debris in surface trawls taken across the North Atlantic Ocean, leading to the first long-term data set on plastic debris (Law et al., 2010). Then, Captain Charles Moore discovered the “Great Pacific Garbage Patch” in 1996 and published the first account of large accumulations of plastic debris in the middle of the North Pacific Subtropical Gyre (Moore et al., 2001). And, in 2004, Richard Thompson coined the term “microplastic” to refer to the ubiquitous small plastic particles (<5 mm in size) found in ocean sediments and surface waters (Thompson et al., 2004). In that same article, Thompson and colleagues made a call for more research on this emerging contaminant, ultimately leading to an exponential rate of increase in scientific evidence regarding the contamination, fate, and effects of plastic debris in the ocean.
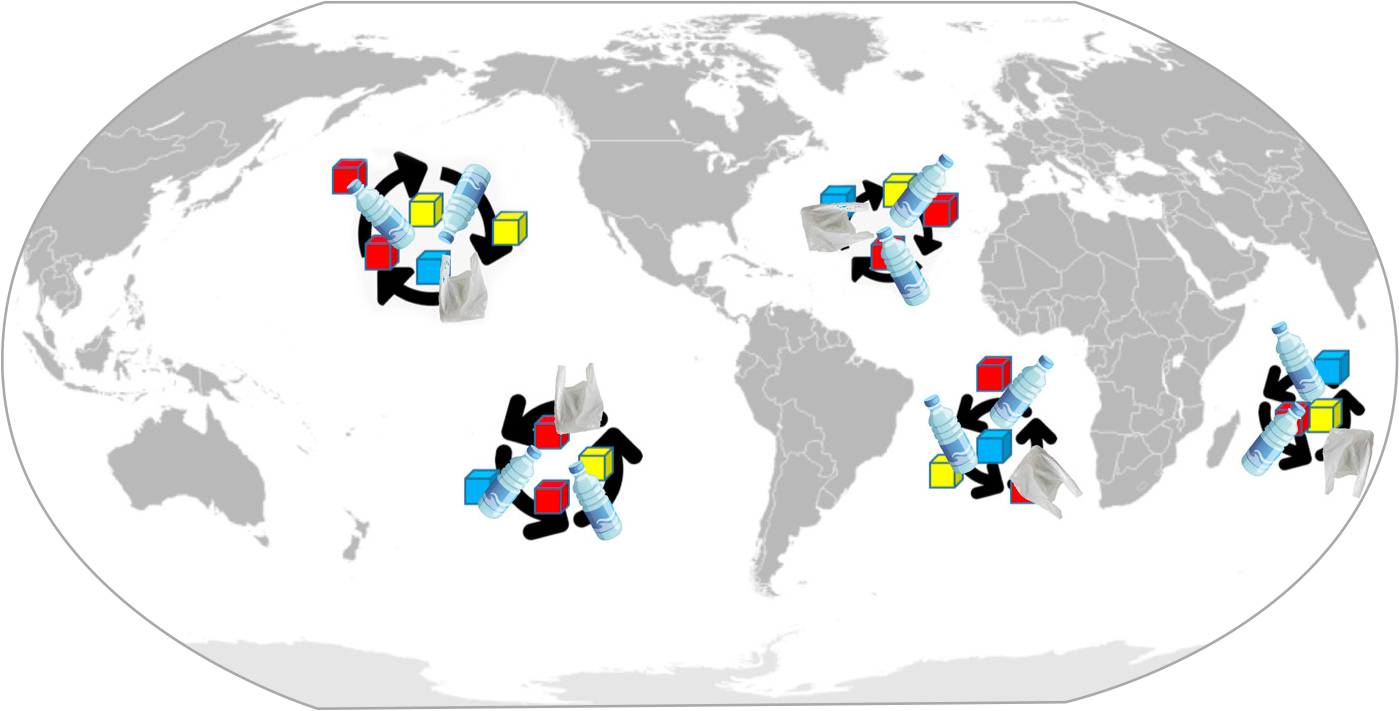
Figure 1. Representation of plastics floating in the five large subtropical gyres centered in the North Pacific, South Pacific, North Atlantic, South Atlantic, and Indian Oceans. > High res figure
|
The history of public awareness around the issue also began in the middle of the ocean. Although the Garbage Patch was discovered nearly two decades prior, an article by Ken Weiss, part of a Pulitzer Prize winning series in the Los Angeles Times, introduced it to the world in 2006 (Weiss, 2006). After this introduction, the Garbage Patch was described again and again as an island of floating plastic litter twice the size of Texas. Intrigued by this idea, and with some disbelief, a group of graduate students led by Miriam Goldstein at the Scripps Institution of Oceanography (SIO) heard Thompson’s call for research and found funding for the first scientific expedition to the Great Pacific Garbage Patch in the summer of 2009.
Aboard R/V New Horizon, a team of graduate students, a videographer, a teacher, and an entrepreneur from an environmental nongovernmental organization called Project Kaisei set off from San Diego to see what they could find (Figure 2a). I was just finishing up my first year of graduate school and was thrilled to have a spot on the ship. Every four hours, we dropped surface-skimming nets into the water to quantify small floating plastic debris, and deck observers worked around the clock to quantify and characterize any large debris. Day after day, there was no sign of a floating island of plastic garbage. Then, on the fourth day, the observers on deck called for assistance. They were using two rulers mounted on the bow of the ship to count debris as it passed. Up to that point, they had logged a buoy, a drink tray, and a fishing net here and there. But then, all of a sudden there were too many pieces of plastic to count, and the two observers needed reinforcements. Looking over the bow of the ship, we saw thousands of little pieces of plastic debris smaller than a pencil eraser (Figure 2b). This was not a garbage patch but rather a soup of microplastic particles (particles <5 mm in size) with large plastic objects here and there.
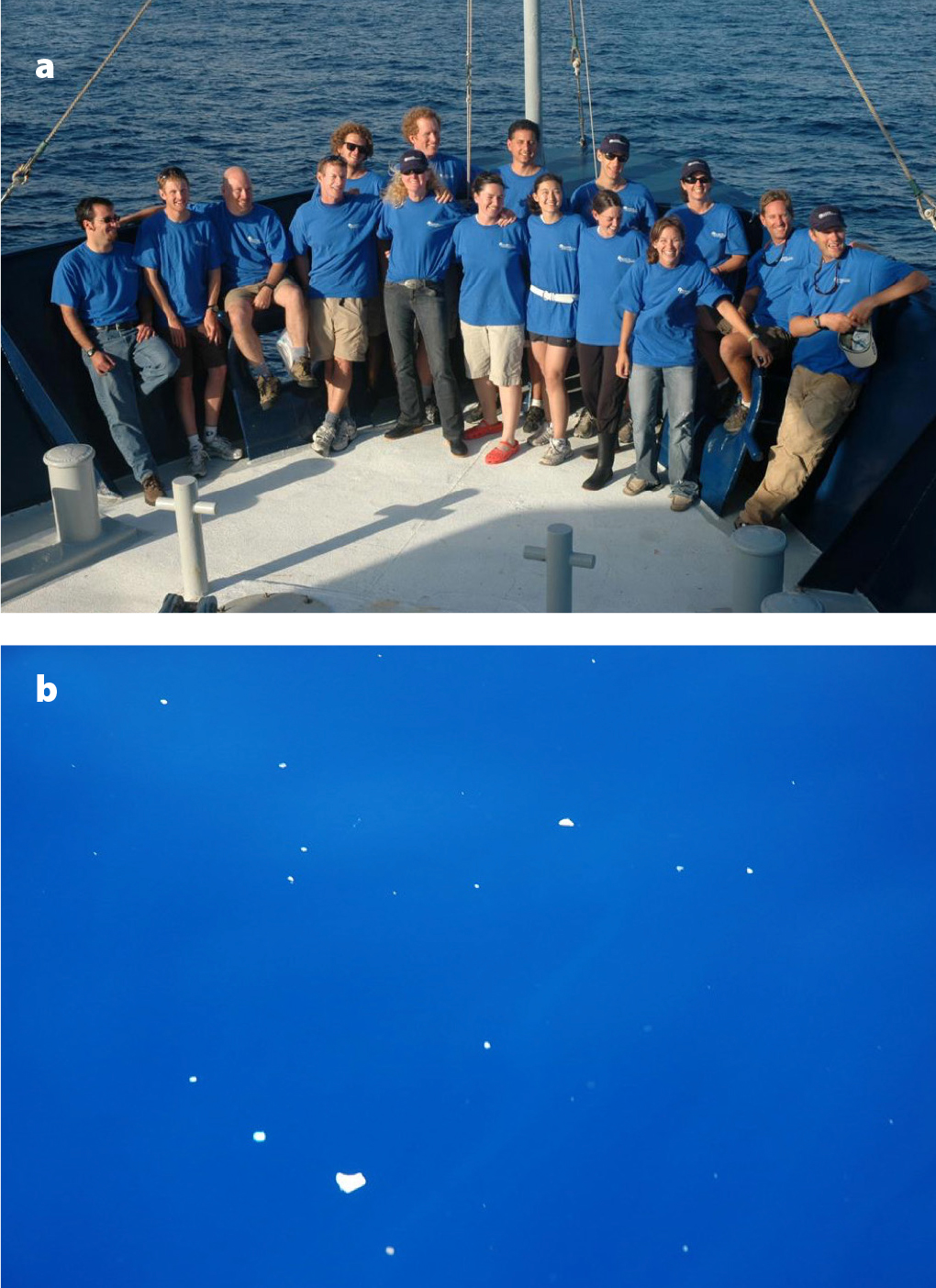
Figure 2. (a) The group of graduate students, other researchers, educators, photographers, and entrepreneurs aboard R/V New Horizon in 2009. (b) The sight from the bow when R/V New Horizon entered the microplastic soup of the Great Pacific Garbage Patch. Photo credits: Scripps Institution of Oceanography. > High res figure
|
Coming back to land, the Scripps researchers aboard New Horizon found microplastics in nearly every sample (Goldstein et al., 2012). This finding—that the majority of plastic pieces were microplastics—demonstrated a need to shift from cleanup to the prevention of plastic emissions from land and maritime sources. The findings also demonstrated a need for more science to better quantify the magnitude of the problem and to better understand the sources, transport, transformation, and impacts of plastics in the marine environment.
A Diverse Size Spectrum: Macro- vs Microplastic
Before diving deeper into the scientific body of work, it is important to spend some time getting to know plastic as the subject. Plastic comes in diverse sizes, shapes, colors, and chemistries (Figure 3). By weight, large plastic debris such as fishing nets make up the greatest percentage of plastic floating in our ocean (Lebreton et al., 2018). However, microplastics make up the most by count. Researchers estimate that there are between 15 and 51 trillion microplastic particles floating at the ocean’s surface (van Sebille et al., 2015), from the poles to the equator. Note that this is just at the surface; researchers have found microplastics in the deepest parts of the ocean (Jamieson et al., 2019) and in Arctic sea ice (Obbard et al., 2014). Although some envision microbeads (the tiny pieces of plastics now banned from face washes) when they think of microplastics, the term microplastic incorporates a diversity of plastic types (Rochman et al., 2019), including those that were produced as microplastics (e.g., microbeads, pre-production pellets often referred to as “nurdles”) and those that are degraded bits of larger plastic products (e.g., tire dust, microfibers, and fragments of consumer products such as bottles, bags, and films). The former are called primary microplastics and the latter are referred to as secondary microplastics. Secondary microplastics are the most common type of microplastic found in the ocean. Both primary and secondary microplastics are important. Microplastics produced from the wear and tear of in-use products are especially abundant, specifically microfibers are some of the most common microplastic morphologies found in global ecosystems (Barrows et al., 2018). This diversity of plastics in the environment, coming from hundreds of different products with diverse polymer backbones and chemical additives, makes this type of pollution complex—different from chemical pollution counterparts such as pesticides or trace metals. Thus, a growing body of researchers investigating plastics in the ocean have posed questions about how these diverse particles interact with physical and biological environments, which has led to an expanded body of knowledge.
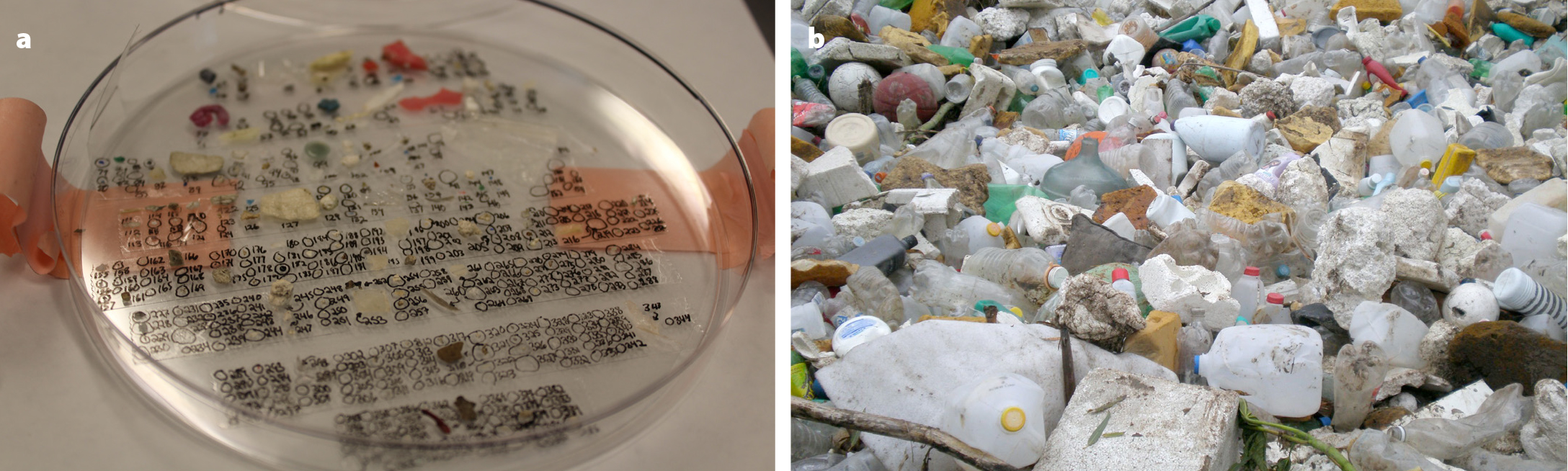
Figure 3. (a) Microplastics collected from a surface water sample taken in San Francisco Bay, California, USA, and mounted on double-sided tape. Photo credit: Cole Brookson, University of Toronto (b) Macroplastics in the riparian zone of the Tijuana River Valley in San Diego, California, USA. > High res figure
|
A Growing Field Spreads Across the Ocean and Toward Dry Land
Our cruise on R/V New Horizon returned from the middle of the North Pacific Ocean to dry land just over one decade ago. Since then, the field has grown tremendously, and the number of scientific papers regarding plastic pollution has increased exponentially (Figure 4). The bulk of the research on plastic pollution is no longer unique to the open ocean, and much of the work now takes place in coastal waters, shallow bays and estuaries, and on beaches—closer to the sources of the pollution.
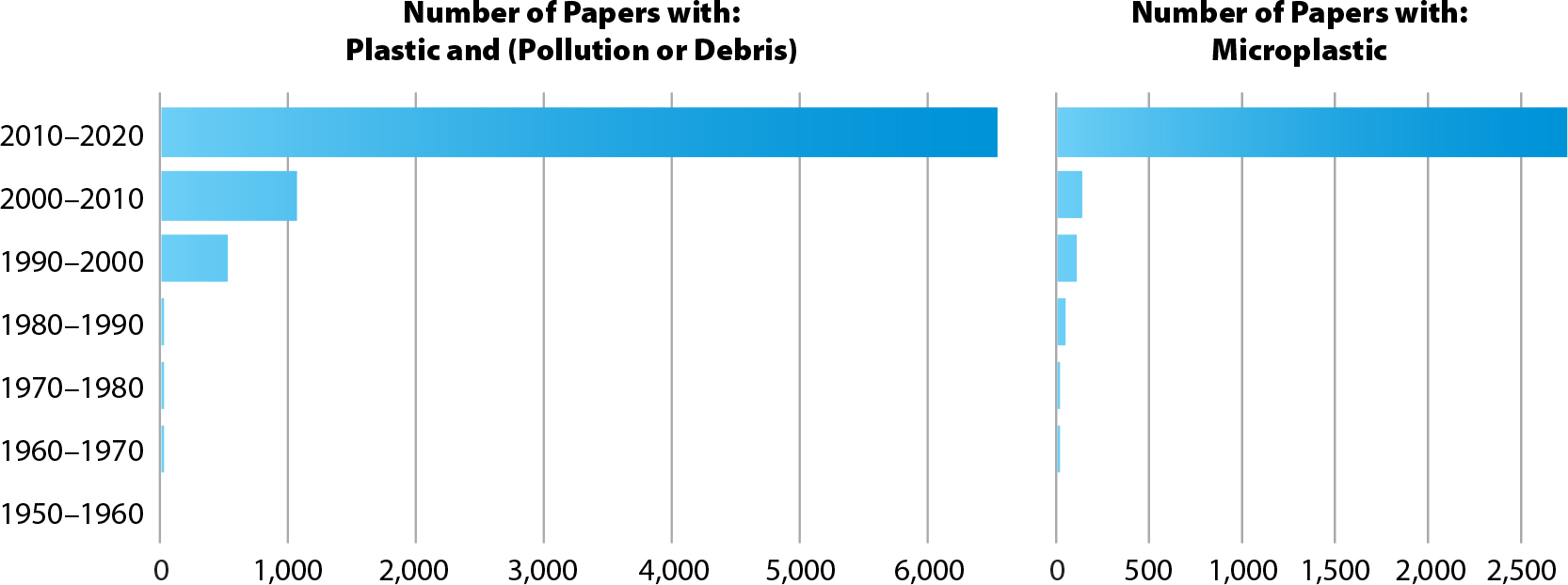
Figure 4. Graph showing the number of papers that have appeared in the Web of Science Core Collection each decade from 1950 through 2020 using search terms “plastic and (pollution or debris)” (left) and “microplastic” (right). > High res figure
|
A growing scientific field has led to an expansion of knowledge followed by further scientific questions. Today, there is no doubt that plastic pollution contaminates the surface of every ocean (van Sebille et al., 2015), the deep sea (Fischer et al., 2015), sea ice (Obbard et al., 2014), and every level of the food web (Gall and Thompson, 2015). Researchers are now working to gain a better understanding of the sources of the contamination, the fates of plastics once they end up in the ocean, the ways in which they transform via physical and biological processes, and how they interact with and alter aquatic ecosystems.
Sources and Pathways of Plastic into the Marine Environment
The sources of plastic pollution are many, and the pathways by which they enter the ocean are equally numerous (Figure 5). Much of the plastic pollution enters the ocean from land, and the rest is thought to enter via maritime activities (e.g., fishing vessels, cruise ships). Sources include mismanaged waste from households, wastewater, and industry (e.g., agriculture, plastic manufacturing). Such mismanaged waste may reach the ocean via littering, being wind-blown from landfills or overflowing garbage bins, natural disasters, accidental spills, or sewage overflows. Rivers are a dominant pathway for plastics to reach the ocean (Lebreton et al., 2017)—plastic debris entering any part of a watershed via stormwater runoff, wastewater effluent, agricultural or industrial runoff has a chance of reaching the ocean.
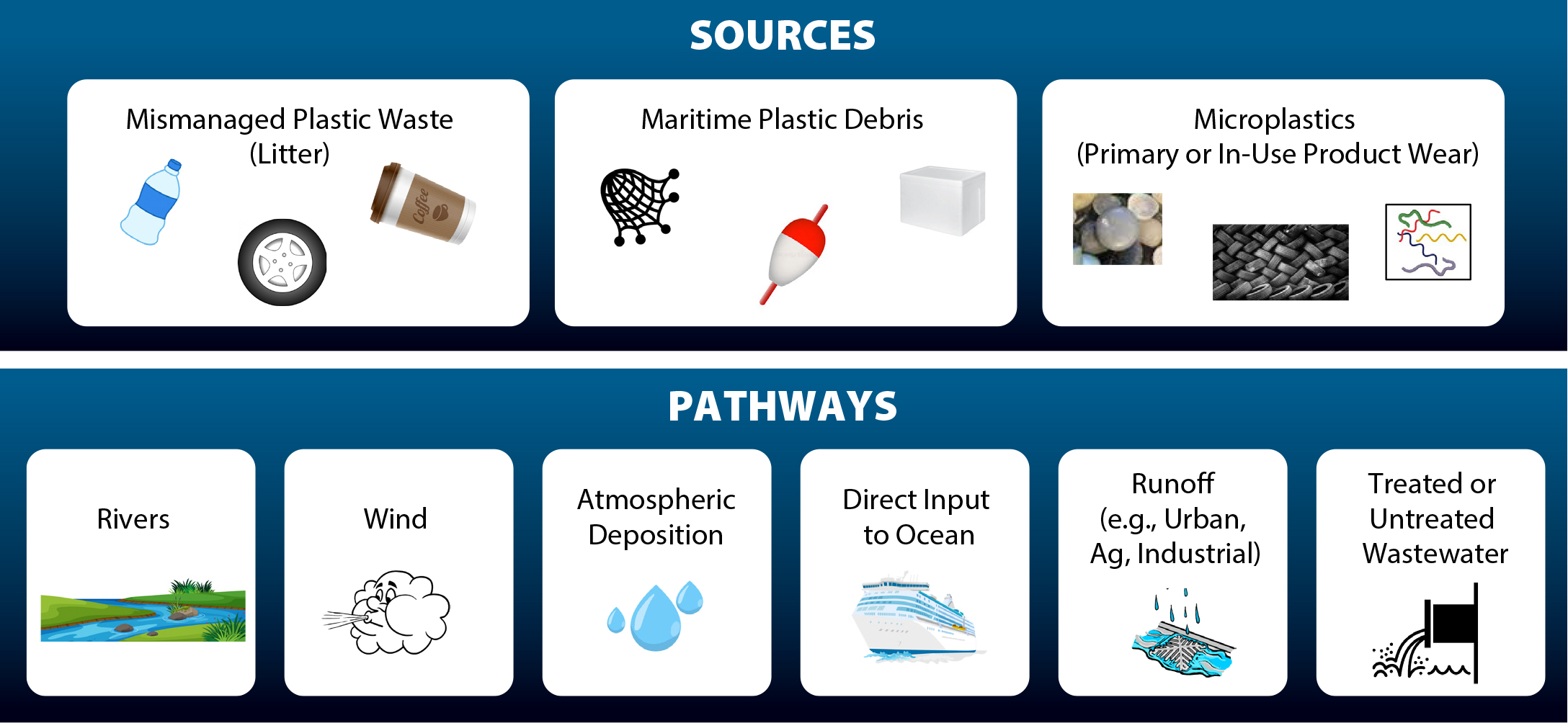
Figure 5. (top) The sources of plastic pollution and (bottom) the pathways by which plastic pollution reaches the ocean. > High res figure
|
Several different emissions models have been created to determine how much plastic waste enters the ocean each year from land (Jambeck et al., 2015; Borrelle et al., 2020; Lau et al., 2020). Jenna Jambeck led the first global emissions model, which was published in the journal Science in 2015. Jambeck et al. (2015) used projected population growth, plastic waste per capita, and proportions of mismanaged waste to estimate the amount of plastic waste entering the environment from each country annually. Using distance from ocean to project how much plastic would reach the ocean, they estimated that 4–12 million tonnes (Mt) of plastic waste entered the ocean in 2010. This model became the baseline. Future models aimed to fine-tune global emissions models using updated or more detailed waste data (Borrelle et al., 2020; Lau et al., 2020), more detailed projections of the amount of plastic debris entering our aquatic ecosystems based on distance from nearest water body (Borrelle et al., 2020; Lau et al., 2020), and by including primary sources of microplastics (Lau et al., 2020). In addition to global models, researchers estimated plastic emissions at a smaller geographic scale (i.e., nationally), with more data to incorporate the trade of waste and illegal dumping (Law et al., 2020) or via a materials flow analysis (Bai et al., 2018). Recognizing that rivers are likely a significant pathway for plastic waste to meet the ocean, other studies have modeled global plastic emissions from major rivers (Lebreton et al., 2017; Schmidt et al., 2017).
Although some of these studies incorporate microplastics into their emissions models (e.g., Lau et al., 2020), most do not. This is likely because the calculated emissions are in mass, and although microplastics make up the largest fraction of plastic in the ocean by count, they make up a relatively small fraction by weight (van Sebille et al., 2015). Moreover, much of the plastic waste that is accounted for in the above emissions models will become secondary microplastics over time via degradation, and including all microplastics in a model could be considered double counting. Still, there are many sources and pathways of microplastics that remain unaccounted for in most of these models because their primary source is not incorporated in statistics for plastic waste. These include microfibers shed from textiles, “dust” released from tires, microbeads in personal care products, plastics used for agricultural applications, and pre-production plastic pellets spilled or leaked from industry. The pathways that bring these microplastics to the ocean include stormwater, agricultural, and industrial runoff, and wastewater (Grbić et al., 2020). A pair of reports estimate that global microplastic emissions from marine paint, cosmetics, road paint, building paint, textiles, pre-production pellets, and tires make up 8%–12.5% of the plastic mass entering the ocean annually (Eunomia, 2016; Boucher and Friot, 2017). One estimate says that tires, pellets, and textiles make up more than 50% of these emissions (Eunomia, 2016), while another estimate says the majority comes from textiles and tires (Boucher and Friot, 2017). Recent work has focused more finely, primarily on microfibers released through washing of textiles, projecting that 0.17 million tonnes of microfibers (Gavigan et al., 2020) are lost to the ocean annually; other work considers sources of microplastics at more regional levels, for example, tire dust in Korea (Lee et al., 2020). These studies suggest the contribution from microplastics is not small, and that further work is needed to better understand microplastic emissions to the global ocean.
Finally, none of these studies include plastic entering the ocean from maritime sources—which has been suggested to be significant (Eunomia, 2016). Emissions from maritime sources include derelict fishing gear, fishing gear lost from aquaculture efforts, wastewater from ships, accidental container spills, and illegal dumping from ships. By mass, mostly due to lost fishing gear, maritime sources likely make up a large proportion of the plastic mass in the ocean—as has been reported in the Great Pacific Garbage Patch (Lebreton et al., 2018). Plastic littered from fishing and shipping vessels has been estimated to make up 14% of annual global plastic emissions (Eunomia, 2016). This includes a predicted loss of roughly 6% of all fishing nets, 9% of all traps, and 29% of all fishing lines to the global ocean annually (Richardson et al., 2019). Further work to estimate maritime sources globally is critical to informing policies aimed at reducing global plastic emissions to the oceans.
Research aimed at determining sources and pathways, which can uncover these unknowns or refine current projections, is essential for defining baselines, assessing risk, and informing mitigation. This may be particularly relevant at more local scales where population size, land use, and local monitoring may help reveal hotspots for risk and key pathways or sources for mitigation. Although this issue is global, mitigation is often implemented at local scales.
The Fate of Plastic in Our Ocean
Researchers are trying to uncover the transport pathways and fate of plastic debris in the ocean as it moves between its physical and biological matrices (or reservoirs). A relevant question first asked in 2004 by Richard Thompson was: “Lost at sea: Where is all the plastic?” (Thompson et al., 2004). To answer this question, we must understand the mechanisms of transport not only within the ocean but also into and out of the ocean. These mechanisms include atmospheric currents and wind, ocean currents, vertical movement in the ocean, marine animal migration, and trophic transfer.
Atmospheric transport is one pathway for microplastics to enter the ocean (Bergmann et al., 2019; Evangeliou et al., 2020). Dris et al. (2015) provided the first evidence of atmospheric transport of microplastics through samples collected in Paris in wet and dry environments, demonstrating that microplastics are a component of dust. This work motivated a deeper understanding of precipitation and dust cycles as global transport pathways for microplastics. Researchers demonstrated that the atmosphere could transport microplastics far from their sources by sampling snow in the Arctic (Bergmann et al., 2019) and collecting wet and dry atmospheric deposition samples in a pristine mountain catchment (Allen et al., 2019). Brahney et al. (2020) examined patterns of atmospheric deposition and found evidence that larger particles originating from local sources get deposited during wet events and that smaller particles originating from faraway sources get deposited during dry events. The latter finding suggested that microplastics are part of global dust cycles. The atmosphere is thus clearly a transport pathway for microplastics to reach the ocean. Recent work also suggests that the atmosphere may also be a pathway for microplastics to exit the ocean via bubble burst ejection and wave action (Allen et al., 2020). As such, mass balance estimates must consider atmospheric transport as pathways both into and out of the ocean.
Currents provide transport pathways for plastics once they enter the ocean. Early studies reporting plastic pollution floating in the subtropical gyres demonstrated the occurrence of long-range transport via wind and surface currents (Moore et al., 2001; Law et al., 2010). Based on a body of work related to understanding surface currents (e.g., Mariano and Ryan, 2007), several researchers used physical oceanographic models combined with empirical data sets to inventory floating plastic debris across the global ocean (Cózar et al., 2014; Eriksen et al., 2014; van Sebille et al., 2015). As these models generally do not incorporate the coastlines and how wind, waves, and surface currents may contribute to beaching, some researchers have discussed and/or tried to estimate the importance of this transport mechanism in removing plastic from the ocean (Lebreton et al., 2012; Kaandorp et al., 2020; van Sebille et al., 2020). In the Mediterranean, beaching was estimated to transport 49%–63% of plastics released into the ocean onto coastlines (Kaandorp et al., 2020).
Plastic debris will eventually leave the ocean’s surface, sinking deeper via several mechanisms that include vertical mixing (Kukulka et al., 2012; van Sebille et al., 2020), sinking of dense debris (Morét-Ferguson et al., 2010), sinking of buoyant debris via biofouling (Kaiser et al., 2017), aggregation with dense organic matter (e.g., marine snow; Porter et al., 2018; Kvale et al., 2020), animal migration (Setälä et al., 2018), and/or integration into dense fecal pellets (Cole et al., 2016; Kvale et al., 2020). Once they leave the ocean’s surface, plastics may be suspended in the water column, be transported via deeper oceanic currents (Kane and Clare, 2019), and/or sink into the sediments (van Cauwenberghe et al., 2013) where they may be buried or resuspended, including via bioturbation (Kane and Clare, 2019). Plastic debris present in deep-sea sediments and organisms (van Cauwenberghe et al., 2013; Woodall et al., 2014; Fischer et al., 2015) demonstrate a vertical flux. Still, few studies have sampled vertical profiles of plastic across the water column (Choy et al., 2019; Li et al., 2020). One such study predicts that the highest concentrations are at the surface, and that concentrations change exponentially with depth (Li et al., 2020). Due to its sheer volume, the water column is likely an underappreciated reservoir of microplastics in the ocean (Choy et al., 2019). Ultimately, the benthos is predicted to be a sink, and several studies predict most plastic debris will be transported from the surface to the deeper ocean and seafloor (Kooi et al., 2017; Koelmans et al., 2017; Kvale et al. 2020).
Finally, the widespread contamination of hundreds of species of wildlife across all trophic levels (Gall and Thompson, 2015), with evidence that plastics can be transported via trophic transfer (Provencher et al., 2019), demonstrates that organisms are also reservoirs and transporters of plastic pollution. Direct ingestion of large plastic debris (Gall and Thompson, 2015) and microplastics has been observed in hundreds of species and across many trophic levels (Gouin, 2020). Other routes of entry include uptake via the gills or indirect ingestion through the consumption of prey (Farrell and Nelson, 2013; Watts et al., 2014). Animals washing up on beaches with large plastics in their gastrointestinal tracts is not uncommon and suggests that relatively large plastics are not egested. Smaller plastics can be egested or excreted, but there is evidence showing that smaller plastics can also translocate, or transfer, outside the gut into different parts of an organism. Field studies report particles beyond the gut of wild-caught animals (Collard et al., 2017, 2018; Abbasi et al., 2018), including particles as large as 438 μm in size in livers and other tissues (Collard et al., 2017). This is fundamental to a larger question, which still remains to be answered, of whether concentrations in tissues increase over time and up food chains (i.e., bioaccumulation and biomagnification). In addition to fate in the food web, animals may be vectors for plastic pollution via migration (van Sebille et al., 2020). This has been demonstrated mainly in birds (Provencher et al., 2018). Although it is clear that animals are a reservoir for plastics in the ocean, the size of this reservoir remains unknown.
Within each reservoir, plastics begin to transform, and these transformations may affect their fate, determining whether they end up in a particular reservoir or whether they are transformed completely. Biofouling has been found to change the density of plastics (Kaiser et al., 2017) in addition to the likelihood that they will be ingested by an animal (Savoca et al., 2017). Degradation processes facilitate the breakup of one piece of plastic into many smaller pieces that may eventually become micro- and nanoplastics (Song et al., 2017). Smaller-sized plastics have different fates in the water column (Kooi et al., 2017) and in an organism (Gouin, 2020). A recent study demonstrated that carbon from microplastics mineralized and utilized by microbes was in turn used by phytoplankton and zooplankton in their cell membrane fatty acids (Taipale et al., 2019). Collectively, a better understanding of the fate and transport of plastic pollution will be facilitated by a better understanding of what has come to be referred to as the “plastic cycle” (Horton and Dixon, 2018; Bank and Hansson, 2019; Rochman and Hoellein, 2020).
Plastic Affects Marine Ecosystems Across All Levels of Biological Organization
As is evident from the section above, plastic pollution has entered every ecosystem, with implications for interactions at every level of biological organization. There is no doubt that large plastic debris can have an impact on wildlife, and compelling evidence suggests that macroplastics are already impacting marine populations, species, and ecosystems (Rochman et al., 2016; Bucci et al., 2020). Studies have reported contamination via entanglement or ingestion in hundreds of species of wildlife. This contamination can lead to laceration of tissues, mortality of an individual organism, declines in population size, and/or changes in the assemblages of species. One recent study published in the journal Science found that plastic debris was correlated with disease in coral reefs (Lamb et al., 2018). In addition, a recent systematic review reports evidence of adverse effects in 23 species of marine mammals, 4 species of turtles, 11 species of birds, 4 species of fish, many species of invertebrates, and one species of algae (Bucci et al., 2020).
The story is more complicated for microplastics. Many studies have tested the effects of microplastics on organisms. Although the results vary, there is irrefutable evidence that microplastics can impact organisms. In laboratory studies, microplastics have been shown to cause a variety of biological effects, including changes in gene expression (e.g., Paul-Pont et al., 2016), inflammation (e.g., von Moos et al., 2012), disruption of feeding behavior (e.g., Cole et al., 2015), decreases in growth (Au et al., 2015), decreases in reproductive success (e.g., Au et al., 2015; Sussarellu et al., 2016), changes in larval development (e.g., Nobre et al., 2015), reduced filtration and respiration rates (e.g., Paul-Pont et al., 2016), and decreased survival (e.g., Au et al., 2015; Cui et al., 2017). Still, while many laboratory experiments find that microplastics can affect the gene expression, growth, reproduction, or survival of an animal, others do not detect any effects and conclude that microplastics have neutral effects (Foley et al., 2018; Bucci et al., 2020). This discrepancy is likely relevant to the complexity and multidimensionality of microplastics as a pollutant—their variation in size, shape, polymer type, and chemical cocktail (e.g., chemical additives and sorbed environmental pollutants).
A closer look across studies suggests that microfibers may be more likely to cause effects than other shapes (Bucci et al., 2020), and that the likelihood for an adverse effect increases with decreasing size of the microplastic (Earn et al., in press). Researchers measuring the toxicity of many types of plastics find a difference among polymers (Zimmermann et al., 2020), suggesting that effects will not be equal among plastic types. Moreover, some plastic types may be more likely to cause effects when their chemical cocktails are more complex. A recent study demonstrated that the mortality of coho salmon in urban streams was due to an additive chemical in the leachate of tire-wear particles (Tian et al., in press). In nature, animals are not exposed to only one plastic size, shape, or type at a time—they are exposed to a mixture (Rochman et al., 2019). Thus, risk assessments need to be relevant to the complex mixture of microplastics, suggesting that novel frameworks are necessary for microplastics. One proposed framework includes a simplification through a three-dimensional probability distribution with size, shape, and density as dimensions (Kooi and Koelmans, 2019). Future frameworks may also include polymer type or chemical mixture.
Still, with the data currently available, thresholds for toxicity can be assessed using existing frameworks. Everaert et al. (2018) combined results from quality laboratory experiments that tested effects to individual endpoints that were relevant to population dynamics (e.g., survival and reproductive output). They included studies that exposed marine animals to plastics of a size range commonly measured in the ocean and that used common buoyant polymer types: polyethylene, polypropylene, or polystyrene. These researchers then synthesized the studies in order to conduct a probabilistic risk assessment to evaluate present and future risks posed by microplastics in the global ocean. They found that unacceptable concentrations range from 8 to 1,490 microplastics per liter, with a mean of 121 particles per liter. Although these concentrations are higher than those found in most parts of the ocean, they are not unheard of. Thus, some of the ocean’s hotspots for plastic contamination (e.g., Mediterranean Sea and Yellow Sea) are currently at risk from microplastic pollution. Everaert et al. (2018) predict the geographic areas where realized risks occur will increase more than twofold by 2050 and one order of magnitude by 2100 if we continue business as usual.
Thus, the clear consensus around the effects of macroplastics, with a burgeoning understanding of the risks of microplastics, inform a need for policies to mitigate plastic pollution now before there is irreparable harm.
Plastic Pollution and Global Change
Due to the ubiquity of plastic debris across our global ocean and freshwater and terrestrial ecosystems (Rochman and Hoellein, 2020), it has been suggested that plastics have a geological cycle that may be used as a signature of the Anthropocene (Zalasiewicz et al., 2016). Moreover, an exponential increase in research on plastic pollution over the last four decades has led to our understanding that plastic pollution is integrated into other fundamental planetary cycles. Plastic debris is involved in the water cycle (Bergmann et al., 2019), the global dust cycle (Brahney et al., 2020), the carbon cycle (Dees et al., 2020; Zhu, in press), and other biogeochemical cycles (Seeley et al., 2020). This integration of plastic into each of these cycles inspires questions about how plastic waste may affect key global processes relevant to biodiversity, ecosystem functioning, biogeochemical cycling, and climate.
Alone, plastic pollution may affect such processes. However, plastic pollution is just one of multiple anthropogenic stressors changing our physical and biological environments. The broad interactions of plastic waste and planetary cycles also bring up questions about how such processes may be affected by the combination of resource extraction, invasive species, climate change, eutrophication, chemical pollution, and plastic pollution, among others. Recognizing that there is strong evidence for plastic debris as a driver of global change, future research should test hypotheses relevant to the mechanisms and extent to which plastic debris, alone and in combination with other drivers of global change, affects our physical and biological planet.
How Did We Get Here?
A 1955 cover of Life magazine celebrated a new lifestyle called “Throwaway Living.” The related article celebrated the rise of single-use products, which included plastic cutlery, plastic straws, plastic plates, and plastic cups (Figure 6). Since then, annual plastic production has increased from 2 Mt in 1950 to 380 Mt in 2015 (Geyer et al., 2017). Today, it continues to rise and is expected to increase by 40% over the next decade. Of course, there are many positive attributes of plastics—they are inexpensive, lightweight, and durable, and their diversity enables a large number of products. Still, some of these positive attributes have also led to a less sustainable usage of these materials (roughly 40% of production is single-use items) and the mismanagement we see today. Of all plastics produced to date, an estimated 9% have been recycled, 12% incinerated, and 79% sent to landfills or littered in the natural environment (Geyer et al., 2017). As mentioned previously, Jambeck et al. (2015) estimate that of the 275 Mt of plastic waste generated in 2010 alone, roughly 8 Mt entered the ocean, becoming plastic pollution. If we continue to do business as usual, annual plastic emissions to the ocean are predicted to increase by an order of magnitude (Jambeck et al. 2015; Borrelle et al., 2020; Lau et al., 2020). These predictions have motivated a global movement with politicians, journalists, and environmental activists agreeing on the need to reduce plastic emissions into the ocean to well below today’s levels.
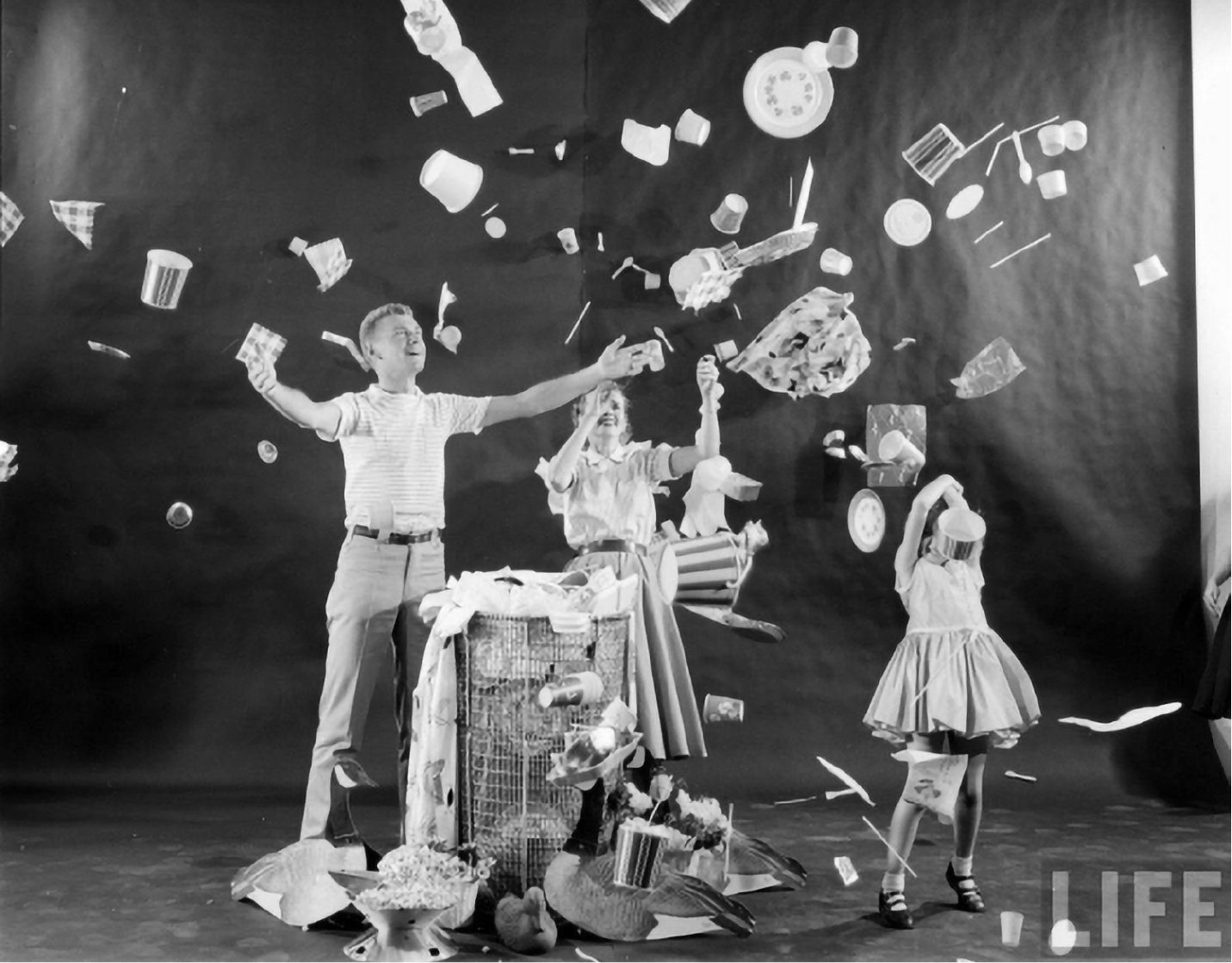
Figure 6. A Life magazine cover celebrating “Throwaway Living” in 1955. > High res figure
|
Stemming the Tide: The Policy Landscape
Today, there is no doubt that anthropogenic debris of all shapes and sizes litters our ocean. It is found in hundreds of species of wildlife, including seafood (Santillo et al., 2017). It is also found in our drinking water (Pivokonsky et al., 2018). We know that plastic pollution harms individual organisms, wildlife populations, and communities (Bucci et al., 2020). These impacts, combined with evidence for accelerating plastic production and leakage into the environment, suggest governments should come together to limit future plastic emissions now, before they transform ecosystems irreparably (Borrelle et al., 2017).
There is no simple solution—and certainly no one-size-fits-all strategy. Unlike CFCs, we cannot simply ban all plastics from production. Diverse policies are necessary at every level of governance. Thus far, we have seen policies enacted at the local level, with single-use product bans for bags, straws, and microbeads. Some cities or states have adopted stormwater and/or sewage bylaws to prevent plastics from entering the water via runoff, and plastic strategies are being considered at the national (Canada) and regional (G7 Ocean Plastics Charter) levels.
Similar to trends in research, international policies relevant to plastic pollution also began in the ocean with MARPOL (International Convention for the Prevention of Pollution from Ships) Annex V that entered into force in 1988. A major part of this annex was a complete ban on dumping plastic from ships. The second international-level policy was the 2011 Honolulu Strategy developed by the US National Oceanic and Atmospheric Administration and the UN Environment Programme (UNEP). It is a planning tool to reduce plastic pollution and its impacts. Then, in February 2017, UNEP announced the Clean Seas global campaign on marine litter, which encourages individuals, industries, and member states to voluntarily commit to actions of their choice, big or small, to reduce plastic pollution. Most recently, in 2019, 187 countries adopted a change to the Basel Convention on the Control of Transboundary Movements of Hazardous Wastes and Their Disposal aimed at restricting international trade in plastic waste. Today, UN Environment is considering an international agreement designed to reduce emissions of plastic pollution well below today’s levels.
Diverse international policies that work in tandem to reduce plastic emissions are necessary. I envision an agreement where countries sign on to a defined reduction target. For example, a country might agree to reduce 25% of its emissions by 2025. To meet reduction targets, each country needs to come up with its own strategies. Because there is no one-size-fits-all solution, each country may take on its own set of unique solutions to reach its target. For example, countries could adopt container deposit schemes to enhance recycling rates, eliminate the use of some single-use plastic items that are unnecessary and not practically recyclable (e.g., straws), improve waste collection and management infrastructure, and agree to market only plastics that are recyclable and/or reusable in their regions. Although policies that mitigate large plastic debris also reduce microplastic debris, we need to make sure microplastics are included in the policy options adopted for plastic pollution. Policies specific to limiting microplastics may include, but are not limited to, leakage standards (e.g., for washing machine effluent, wastewater, stormwater), requiring filters on washing machines to trap microfibers, and use of bioretention cells (or rain gardens, depressed areas in landscapes that collect rainwater from roofs, driveways, or streets and allow it to soak into the ground that may be planted with, for example, grasses and flowering perennials). These policies may also include increasing industry participation in Operation Clean Sweep, a voluntary initiative to reduce resin pellet loss, and extending this model to textiles, material innovation, and microbeads. For some countries, particularly in the developing world, aid is necessary to build new infrastructure for waste. These countries need access to a global fund similar to the United Nations Framework Convention on Climate Change’s climate fund. To build this fund, an extended producer responsibility program could be implemented. If the fund pulled in one penny for every pound of plastic produced, it would increase by over $6.8 billion per year. Countries would report annually on their successes in measuring the reduction of plastic emissions, ensuring that signatories reached their goals.
A Path Forward
Today, scientists estimate that more than 24 million tonnes of plastics enter aquatic ecosystems, including our ocean, each year (Borrelle et al., 2020). It will not be simple to reduce emissions well below this value. This issue is complex. The sources of plastics entering the environment are diverse as are the types of plastics we produce, sell, and find in nature. The ecosystems and organisms this pollution contaminates are also diverse, and as a consequence, the solutions need to be diverse. We need a toolbox of solutions that includes plastic reduction, the building of a circular economy, improved waste management systems, innovation that results in new materials and technologies for prevention, as well as cleanup, outreach, and education. We also need everyone working together, including the plastic industry, waste managers, the public, scientists, and all levels of government from all over the world.
The first decade of my scientific career has been rewarding—I have had the opportunity to grow with a burgeoning field of research. In parallel, I have witnessed our science being used to inform the policymakers planning for positive change. The second decade of my career will be filled with deeper scientific questioning to better understand this diverse contaminant. Still, we have learned a lot, and my hope for this next decade is that we also use the knowledge from the decade past to realize global goals for reduced emissions of plastics into the ocean to protect it for people, wildlife, and the planet, and in recognition of the many heroes of our ocean past, including Dr. Roger Revelle.
Roger Revelle
For almost half a century, Roger Revelle was a leader in the field of oceanography. Revelle trained as a geologist at Pomona College and the University of California, Berkeley. In 1936, he received his PhD in oceanography from the Scripps Institution of Oceanography. As a young naval officer, he helped persuade the Navy to create the Office of Naval Research to support basic research in oceanography. He served for 12 years as the Director of Scripps where he built up a fleet of research ships and initiated a decade of expeditions to the deep Pacific that challenged existing geological theory.
Revelle’s early work on the carbon cycle suggested that the sea could not absorb all the carbon dioxide released from burning fossil fuels. He organized the first continual measurement of atmospheric carbon dioxide, an effort led by Charles Keeling, resulting in a long-term record that has been essential to current research on global climate change.
In 1957, Revelle became a member of the National Academy of Sciences to which he devoted many hours of volunteer service. He served as a member of the Ocean Studies Board, the Board on Atmospheric Sciences and Climate, and many committees.