Historical Context
Trace metals in natural waters exist in various physicochemical forms that differ in size (dissolved, colloidal, particulate), redox states, and complexes with molecules (organic or inorganic ligands). These forms, or species, of trace metals dictate their solubility, reactivity, and bioavailability to microorganisms. Many of the trace metals that are dissolved in seawater have low inorganic solubility, and speciation is defined by the relative contributions of their inorganic and organic forms. Measuring the chemical speciation of trace metals that are dominated by organic complexation, such as iron, copper, nickel, cobalt, and zinc, thus requires the characterization of metal-binding organic ligands, which comprise a small fraction of the much larger, heterogeneous, and largely uncharacterized pool of dissolved organic matter (DOM) that exists in seawater (Gledhill and Buck, 2012; Dittmar and Stubbins, 2014).
It has been 40 years since the first dissolved organic speciation measurements were made in open ocean waters (van den Berg, 1984). Early measurements showed that organic ligands complexed large portions of the dissolved pool of many trace metals, up to 99.9% in the case of iron (see Gledhill and Buck, 2012, and references therein). The importance of ligands in controlling dissolved iron concentrations was recognized in the earliest efforts to model dissolved iron cycling in the ocean (Johnson et al., 1997). Since these early studies, it has been widely recognized that ligands play an important role in the cycling of trace metals (Figure 1).
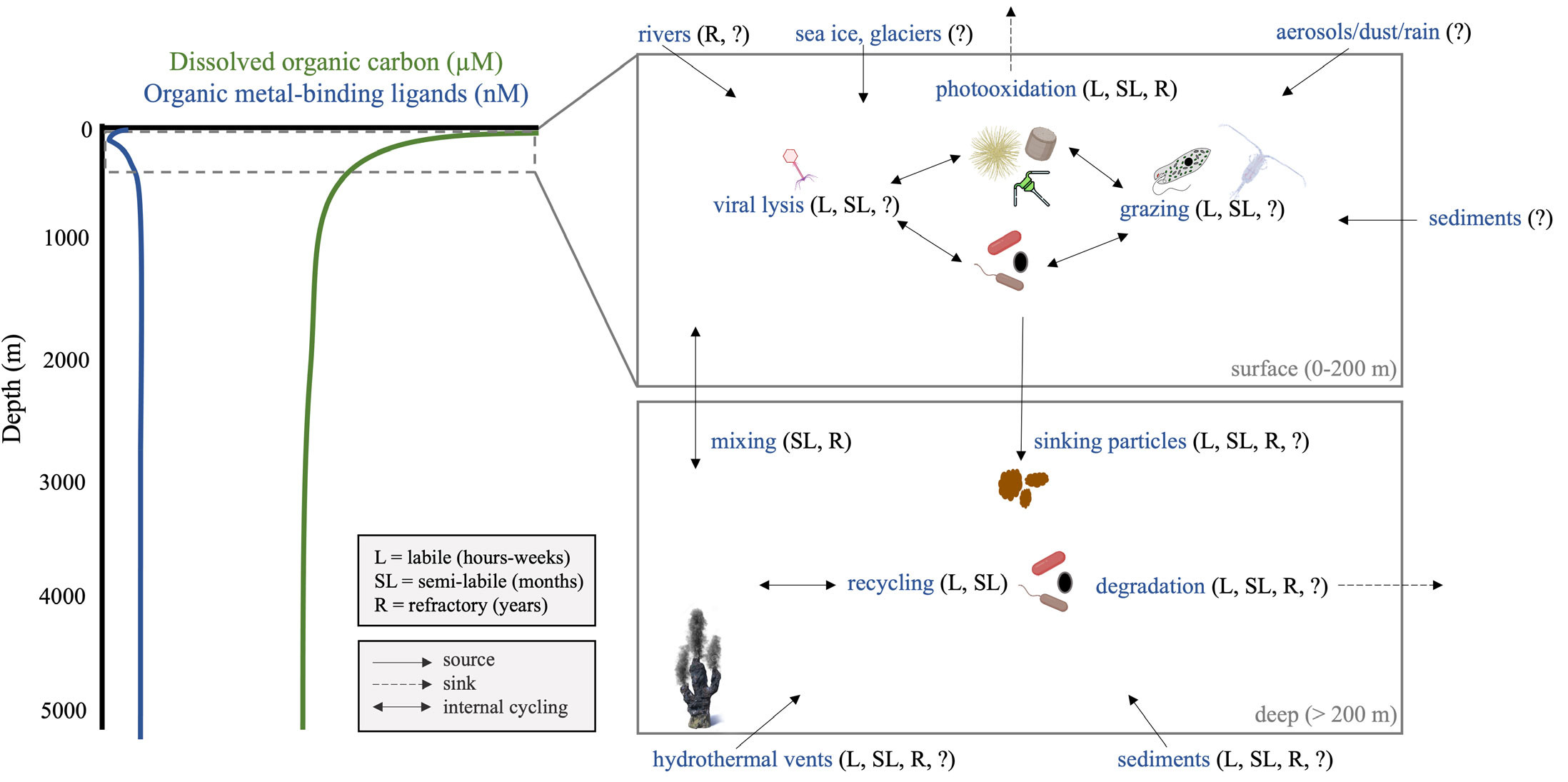
FIGURE 1. Key biogeochemical processes impacting metal-binding ligand production, cycling, and degradation in seawater. L, SL, and R refer to labile (turnover time of hours to weeks), semi-labile (months), and refractory (years) materials, respectively, while arrows denote sources, sinks, and internal cycling. Question marks highlight prevailing knowledge gaps. > High res figure
|
On short timescales, observed dissolved metal concentrations in seawater greatly exceed inorganic solubility limits because organic ligands prevent the precipitation and abiotic scavenging of metals. On longer timescales, ligands facilitate the exchange of metals between the dissolved, colloidal, and particulate phases, stabilize metals for long-distance transport, and govern the overall oceanic inventory of metals in seawater. As a result, the importance of understanding the composition, bioavailability, and internal cycling processes of organic ligands was highlighted in the GEOTRACES Science Plan (GEOTRACES Planning Group, 2006).
GEOTRACES survey and process study expeditions have enhanced our understanding of metal speciation by identifying important regional and basin-scale features in ligand distributions, particularly for iron. However, recent advances have also drawn attention to the importance of inorganic forms of metals (M') and the difficulties in relating organic speciation (ML, where M is a metal and L represents an organic ligand) to metal bioavailability. Here, we focus on the key insights that have been garnered on metal speciation since the conception of the GEOTRACES Science Plan, with a focus on dissolved organic iron-binding ligands. We highlight how the broad spatial distribution of measurements is allowing us to unravel regional patterns in ligand distributions, how our understanding of ligands relates to the wider DOM pool, and how metal speciation has been incorporated into regional and global ocean scale biogeochemical models. Finally, we consider the challenges of understanding metal bioavailability and close with a look toward the future of understanding metal speciation and metal bioavailability in the new GEOTRACES era focused on process studies.
Advancements Across Temporal and Spatial Scales
Implementation of the international GEOTRACES program has greatly increased the resolution of metal speciation data from the ocean, particularly for iron, due to its recognized role as a limiting nutrient across over a third of the ocean surface (J.K. Moore et al., 2001). The number of measurements of dissolved iron-binding organic ligands has increased 20-fold since the launch of the GEOTRACES field program in 2010 (Figure 2; Supplementary Table S1). Prior to GEOTRACES, most iron-binding ligand data were focused in the Atlantic and within the upper ocean; now, full depth profiles are available from every major ocean basin, although there is still relatively limited data from the Indian Ocean. There has also been an increase in the number of speciation studies on other bioactive trace metals, including copper, cadmium, zinc, and cobalt.
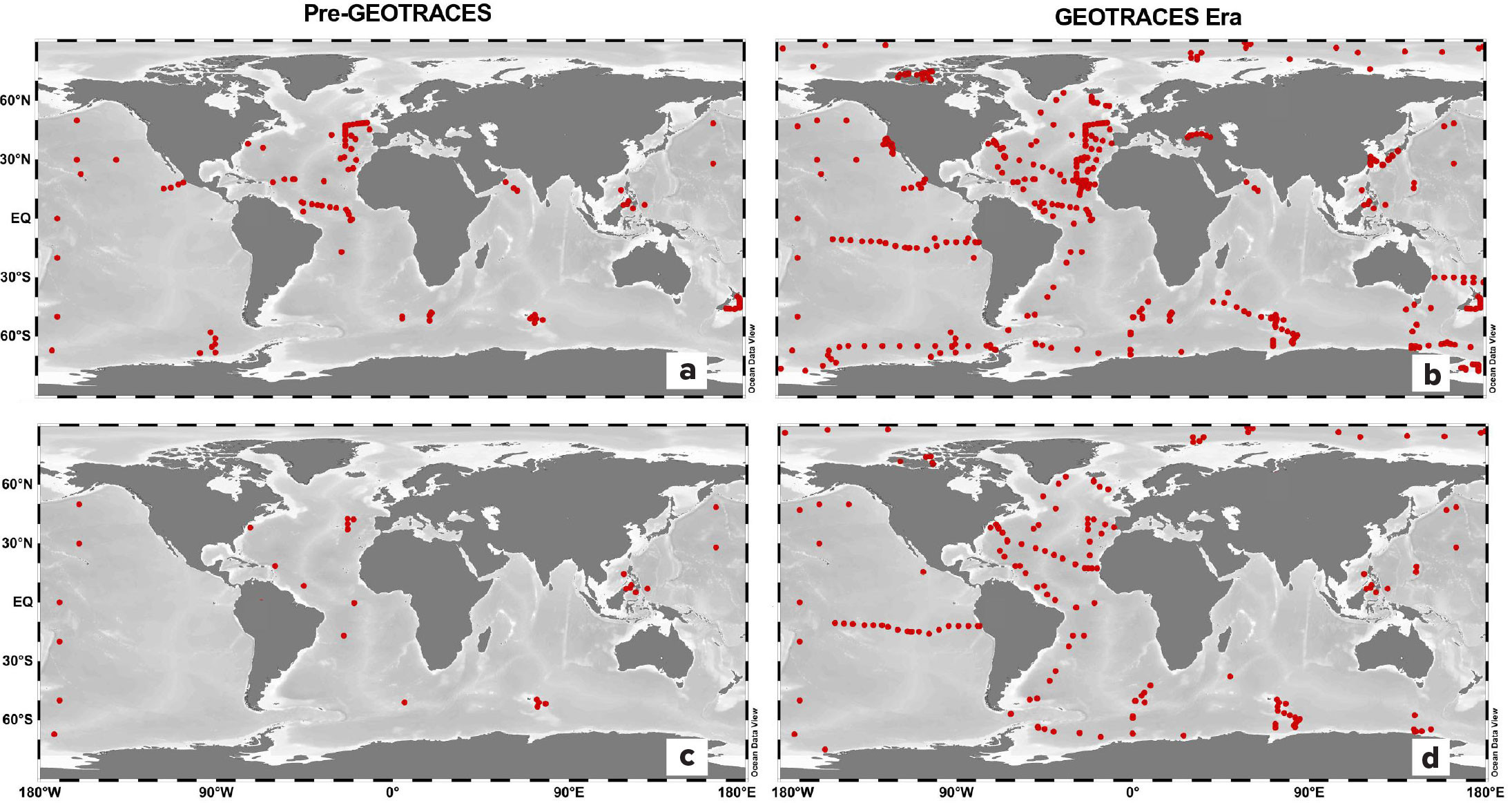
FIGURE 2. Dissolved iron-binding ligand data in the pre- and post-GEOTRACES eras. Red dots mark locations for iron-binding ligand data published before 2005 (a), and after 2005 (b). (c) Dots indicate locations where iron-binding ligand data were collected below 1,000 m before 2005, and (d) after 2005. References for each study are found online in Table S1. > High res figure
|
Intercalibration of Methods to Characterize Metal-Binding Organic Ligands
Competitive ligand exchange-adsorptive cathodic stripping voltammetry (CLE-AdCSV) is by far the most commonly used method to determine the concentration and metal binding capacity of organic ligands in seawater (see Gledhill and Buck, 2012, and Moffett and Boiteau, 2024, and references therein). This approach provides the concentration of the ligands and their average binding strength for a specific metal within a detection window, or across multiple analytical windows (Moffett et al., 1997; Bruland et al., 2000). These ligand characteristics allow for broad operational classification of ligands based on their conditional stability constants for iron (Gledhill and Buck, 2012) and for copper (Bruland et al., 2000; Ruacho et al., 2022) into strong (L1), intermediate (L2), and progressively weaker (L3, L4…) ligand classes. For iron, these logKFe' values are operationally defined as L1 >12, L2 = 11–12, and L3 <11; for copper, they are defined as logKCu2+ values of L1>14, L2 = 10–14, and L3<10. Such classifications enable studies to define ligand characteristics across methods and provide insights into components of the ligand pool and their roles in influencing metal speciation. A modified version of the CLE technique using a solvent extraction-based approach has also recently been developed (Moriyasu and Moffett, 2022) and used to determine the inert copper fraction in the GEOTRACES GP15 section of the equatorial and North Pacific (Moriyasu et al., 2023). The binding strength of some metals can also be characterized by pseudovoltammetry and kinetics-based experiments in which conditional stability constants determined by kinetic experiments with metal additions provide excellent agreement with those from CLE-AdCSV titrations, supporting both approaches (Luther et al., 2021, and references therein).
In preparation for GEOTRACES basin-scale surveys, an international intercalibration of commonly applied CLE-AdCSV approaches for iron- and copper-binding organic ligand measurements was conducted in samples collected from three ocean depths in the North Pacific (Buck et al., 2012), building on previous work for copper (Bruland et al., 2000). This effort led to specific recommendations for the collection and storage of organic ligand samples (https://www.geotraces.org/methods-cookbook/), and for the application of these CLE-AdCSV methods to determine comparable results for the characteristics of both iron- and copper-binding organic ligands in open ocean waters (Buck et al., 2012). The two CLE-AdCSV methods that have been intercalibrated most extensively employ the added ligands salicylaldoxime (SA) or 2-(2-thiazolylazo)-p-cresol (TAC), both of which were successfully vetted in the original method development against model ligands that included the siderophore desferrioxamine B (Rue and Bruland, 1995; Croot and Johansson, 2000). Each method has challenges; the TAC method may underestimate the contributions of humic-like ligands (Slagter et al., 2019; Ardiningsih et al., 2021), and thus the total iron-binding ligand pool (Gerringa et al., 2021), while a loss of iron-SA complexes during the equilibration period can lead to an overestimation of ligand concentrations (Gerringa et al., 2021; Mahieu et al., 2024); however, this can be avoided by conditioning the titration vials with repeated exposure to anticipated iron additions (Rue and Bruland, 1995; Buck et al., 2007; Mahieu et al., 2024).
Independent application of these methods by analysts from the US and Dutch GEOTRACES programs to depth profiles at the Bermuda Atlantic Time-series Study (BATS) crossover station in the Atlantic Ocean showed that the two methods provide excellent agreement on the concentrations and conditional stability constants of stronger iron-binding organic ligands (Buck et al., 2015, 2016; Gerringa et al., 2015). Characterizing weaker iron-binding organic ligands is still a challenge, as not all CLE-AdCSV methods can detect humic-like iron-binding organic ligands (Slagter et al., 2019; Ardiningsih et al., 2021). Despite the various analytical challenges highlighted here, GEOTRACES activities are leading to the standardization of procedures and improved reproducibility of ligand datasets, and characterizing metal-binding ligand concentrations has proven critical to advancing understanding of iron cycling in the global ocean (Tagliabue et al., 2023).
Altogether, initial intercalibration and method assessment efforts, combined with parallel advancements in voltammetric data manipulation and interpretation (Wells et al., 2013; Omanovic et al., 2015; Pizeta et al., 2015), achieved through parallel efforts of SCOR working group 139 associates (https://scor-int.org/group/139/), helped pave the way for the high throughput analyses of metal-binding organic ligands needed to characterize metal speciation in the ensuing GEOTRACES basin-scale surveys and process studies. To date, metal-binding organic ligands have been characterized across GEOTRACES section cruises in the Atlantic, Pacific, Arctic, and Southern Oceans, and the Mediterranean and Black Seas (see Table S1 for iron references and the section below on the Spatial Distribution of Metal-Binding Ligands for other metals). Continued method assessments and intercalibration efforts, however, are highly encouraged. The GEOTRACES website (https://www.geotraces.org/about-intercalibration/) provides guidelines for interested analysts to initiate intercalibration exercises as well as contact information for elemental coordinators, and the GEOTRACES cookbook provides useful information on sample handling protocols for metal-binding ligands that emerged from early intercalibration efforts (Cutter et al., 2017).
Spatial Distribution of Metal-Binding Ligands
The compilation of metal-binding ligand measurements produced by international analysts participating in GEOTRACES sections has revealed consistent features in global distributions of ligands and fueled new insights into the cycling of metals, particularly iron, in the ocean. One of the earliest findings during GEOTRACES was that the strongest iron-binding ligands are present throughout the entire water column, especially in the Atlantic (Buck et al., 2015, 2018; Gerringa et al., 2015). These ligands have relatively constant concentrations with depth, and excess ligand concentrations are often highest in surface waters, reflecting ligand production associated with primary productivity (see Gledhill and Buck, 2012, and references therein). However, at the global-ocean scale, there is a progressive loss of stronger iron-binding ligands and of ligands not already bound to iron (so-called “excess ligands”) at depth with water mass age and between the deep waters of the Atlantic and Pacific (Thuróczy et al., 2011; Gerringa et al., 2015; Buck et al., 2018). Patterns of ligand distributions in different water masses have also been revealed by basin-scale studies. For example, Buck et al. (2018) noted that excess iron-binding ligand concentrations and binding strength were higher in water masses originating in the Southern Ocean, such as in Circumpolar Deep Water and Antarctic Intermediate Water. These observations suggest that some portion of the ligand pool might be set in regions of intermediate and deep water formation with important implications for dissolved iron cycling (Buck et al., 2018).
Ligand pool composition varies spatially and with depth due to local sources, sinks, and cycling processes. Ligands are produced and released by microorganisms to compete for limited trace metal resources, reduce trace metal toxicity, solubilize particulate phases of trace metals originating from sources such as atmospheric deposition and hydrothermal vents, and recycle metals from decaying biomass. Speciation studies (Table S1) have identified margin sediments as an important source of ligands in both oxic and low oxygen regions, while some regions, such as the Arctic Ocean, are heavily influenced by ligands originating from terrestrial material. Active bacterial remineralization of marine particles at depth also releases an array of metal-binding ligands of various strengths (Boyd and Ellwood, 2010; Velasquez et al., 2016; Bundy et al., 2018; Whitby et al., 2020a). Atmospheric deposition may have a large influence on the composition of metal-binding ligand pools in areas of the ocean that receive large seasonal or episodic inputs of dust, such as the equatorial Atlantic, North Pacific, and Indian Oceans (Mahowald et al., 2005), as dust input can lead to higher iron binding ligand concentrations (Wagener et al., 2010; Wuttig et al., 2013) and stimulate siderophore production (Basu et al., 2019; Park et al., 2023). Speciation measurements around hydrothermal vents have revealed the importance of ligands in stabilizing hydrothermal supplies of dissolved metals for long distance transport (Sander and Koschinsky, 2011; Buck et al., 2015; Resing et al., 2015; Wang et al., 2019, 2021). This may reflect active production of ligands by hydrothermal plume communities to manage or access metals, in particular iron (Hansen et al., 2022). Sea ice melt may also contribute organic ligands to seawater (Lannuzel et al., 2015; Genovese et al., 2018; Arnone et al., 2023, 2024).
Although important insights into the sources and sinks of ligands have been gained through GEOTRACES spatial studies, fewer data are available on the temporal cycling of various components of the ligand pool. A study in the oligotrophic Pacific at the Hawai‘i Ocean Time-series site found that iron-binding ligand concentrations in the euphotic zone can change on the order of days (Fitzsimmons et al., 2015b), while lab-based studies have demonstrated that some strong iron-binding ligands have very slow dissociation kinetics (Boiteau et al., 2018). However, these experiments are more representative of the residence time of the iron complex than of the ligands themselves. Ligands may have much longer residence times; another study estimated a residence time of around 750 years for organic ligands in North Atlantic Deep Water, 2.5 to 4 times larger than the estimated residence time of dissolved iron within the same water mass (Gerringa et al., 2015). The Arctic Ocean may be a potential source for these refractory ligands, as it has a strong terrestrial signature in the upper water column, particularly within the Transpolar Drift, which transports material of riverine origin across the basin with signature high concentrations of iron- and copper-binding organic ligands relative to surrounding waters (Slagter et al., 2019; Arnone et al., 2023). This poses questions about the importance of regional differences in the sources and sinks of ligands and how such differences may impact metal speciation and bioavailability. Overall, the temporal scale dynamics of ligands likely depends heavily on the types of ligand and local biogeochemical processes (Figure 1).
There are a limited but growing number of studies regarding the organic complexation of other metals. A recent review summarizes advances in our understanding of the copper-binding ligand pool in detail, including open ocean speciation measurements from the Atlantic, Pacific, and Southern Oceans, as well as advances in modeling copper ligands (Ruacho et al., 2022, and references therein). More recently, copper speciation has also been measured in the Arctic (Arnone et al., 2023, 2024). Methods complementary to CLE-AdCSV have provided further information on copper lability (Moriyasu and Moffett, 2022; Moriyasu et al., 2023), and have allowed us to interrogate ligand sources (Karavoltsos et al., 2013; Nixon et al., 2019, 2021) as well as to study the uptake of ligand-bound copper (Semeniuk et al., 2015). Copper-binding ligands (along with those for nickel) have also been characterized at the molecular level (Boiteau et al., 2016b; Babcock-Adams, 2022).
GEOTRACES studies have also allowed us to better understand the marine cobalt cycle across different ocean basins, including the Atlantic, Pacific, Arctic, and Mediterranean (Noble, 2012; Hawco et al., 2016; Dulaquais et al., 2017; Noble et al., 2017; Bundy et al., 2020; Chmiel et al., 2022). Applications of CLE-AdCSV methods have found that dissolved cobalt exists in both labile (free Co(II) and weakly bound Co) and strong ligand-bound forms, and that most of these organic ligands strongly bind to Co(III), as Co(II) is outcompeted by other divalent trace metals like nickel and copper (Baars and Croot, 2015). Cyanobacteria or phytoplankton may be a source of cobalt ligands in the surface ocean (Saito et al., 2005; Baars and Croot, 2015), while cyanocobalamin and other cobalamin analogues have been suggested as potential cobalt ligands because of their extremely high conditional stability constants (Ellwood and van den Berg, 2001).
Zinc-binding ligands have been found to be oversaturated in high latitude waters in the Southern Atlantic and in the western subarctic North Pacific due to high dissolved zinc concentrations (Baars and Croot, 2011; T. Kim et al., 2015). In contrast, zinc ligands were generally in excess of dissolved zinc in the Tasman Sea (Sinoir et al., 2016). Some zinc-binding ligands are likely derived from phytoplankton production, as suggested by high correlation between total zinc ligands and biomarker pigments (T. Kim et al., 2015; Sinoir et al., 2016). One study suggested that degradation products of bacteria and phytoplankton, such as zinc-chlorophylls, porphyrins, and proteins, may act as zinc-binding ligands based on the formation rates of these complexes (Baars and Croot, 2011), but these ligands have not been directly chemically characterized to date.
For cadmium, over 70% of the dissolved form is bound to strong organic ligands in surface waters of the North Pacific, subantarctic Pacific, and Atlantic (Bruland, 1992; Ellwood, 2004; Baars et al., 2014). One study found the highest concentrations of cadmium-binding ligands were in areas with high chlorophyll, suggesting a biological source, while weak cadmium-binding ligands may be derived from marine humic material (Baars et al., 2014).
Linking Metal Speciation with Dissolved Organic Matter
Marine DOM is a heterogeneous mixture of organic molecules, from well-defined biomolecules synthesized for specific purposes to myriad degradation products that have been biotically and abiotically transformed. A variety of metal-binding functional groups exist within DOM, including amines, thiols, phenols, and carboxylates. However, dissolved organic carbon (DOC) concentrations register at 34–70 µM throughout most of the ocean (Dittmar and Stubbins, 2014), over four orders of magnitude higher than typical trace metal and metal-binding organic ligand concentrations. This suggests that while many DOM components interact with metals to some degree, only a minor fraction of the DOM pool has a great impact on metal speciation, distribution, and cycling.
While operationally characterizing ligands based on their conditional stability constants has proven insightful in understanding processes that influence metal speciation and cycling at the global scale (Gledhill and Buck, 2012; Buck et al., 2018), significant progress has also been made in identifying specific ligands that contribute to the metal-binding ligand pool in seawater, in particular for iron. These insights are helping trace metal chemists connect our understanding of metal speciation to the wider DOM pool. For example, short-lived or semi-labile ligands in productive upper waters may be different from the refractory ligands that transport metals long distances and maintain the deep ocean metal inventory (Figure 1), but all are part of the DOM pool. Concurrently, ligands that are considered refractory when at depth may be photosensitive and labile when upwelled (Hassler et al., 2020).
A widely recognized, strong iron-binding ligand group for iron are siderophores, small molecules produced by marine microbes to mediate iron uptake (Vraspir and Butler, 2009). Rue and Bruland (1995) made some of the first measurements of iron-binding ligands in seawater using voltammetry. They noted that the binding strengths of the strongest ligand class were remarkably similar to siderophores and hypothesized these compounds to be important iron ligands. Advances in mass spectrometry have since allowed direct quantification and identification of siderophores from the open ocean at depths ranging from the surface to at least 1,500 m (see Moffett and Boiteau, 2024, and references therein). However, it is unclear if siderophores are actively released at these depths and only in response to iron limitation, or if they can be long-lived molecules.
GEOTRACES transects have revealed that siderophores are widespread across the ocean and that their composition is diverse and varies across ocean biomes (Boiteau et al., 2016a). Amphiphilic siderophores with fatty acid tails that enable them to be tethered to cell membranes are particularly high in chronically iron-limited surface waters as well as mesopelagic waters with high nitrate and low dissolved iron, reflecting their role in facilitating iron dissolution and uptake (Boiteau et al., 2016a; Bundy et al., 2018; Park et al., 2023). Some siderophores have also been observed in environments with high iron concentrations, either during particle remineralization experiments (Velasquez et al., 2016; Bundy et al., 2018) or within waters associated with benthic resuspension layers (Boiteau et al., 2019), or nearshore oxygen minimum zones (L.E. Moore et al., 2021), suggesting that although iron concentrations are high in some areas, it may not be in a sufficiently bioavailable form to sustain microbial metabolism. Siderophores thus far appear to be minor contributors to the total iron-binding ligand pool in seawater, as they are present in picomolar concentrations compared to the nanomolar concentrations of the total ligand pool (Bundy et al., 2018). However, siderophore characterization relies on the efficiency of methods for extracting these compounds from seawater, and methodological advancements are still needed to capture the most polar siderophores.
Despite their low concentration, siderophores are likely important conduits by which microbial communities regulate iron cycling. Based on measured uptake rates from incubation experiments, siderophores are expected to fall within the labile fraction of the DOM pool (Figure 1). Siderophores produced in response to iron deficiency can accelerate the dissolution of iron from particles, minerals, and colloids (Borer et al., 2005; Kraemer et al., 2005). Furthermore, siderophores represent a reservoir of iron only available to a subset of the microbial community that is equipped with siderophore-specific uptake systems (Lis et al., 2015a; Kramer et al., 2020) and thus can mediate synergistic or antagonistic interactions between different members of the microbial community. Iron complexation by siderophores was shown to decrease iron bioavailability 1,000-fold compared to Fe' (unchelated iron) in many phytoplankton taxa lacking siderophore transporters (Lis et al., 2015a, 2015b). However, growing evidence suggests that marine photoautotrophs adapted to iron-starved conditions may benefit from siderophores produced by other microbes (Basu et al., 2019; Coale et al., 2019; Hogle et al., 2022). Additionally, photoreactions of siderophores can lead to iron reduction and release and the production of weaker ligands (Barbeau et al., 2001), which may increase the pool of bioavailable iron for the wider microbial community (Shaked and Lis, 2012; Lis et al., 2015b; Mellett et al., 2018; Manck et al., 2022).
Much of the labile and semi-labile DOM pool is composed of acidic sugars, exopolysaccharides, and exopolymeric substances (EPSs), some of which act as weaker metal-binding ligands for metals such as iron and copper (Plavšić and Strmečki, 2016; Hassler et al., 2017). Incubation studies have demonstrated that EPSs increase iron solubility and uptake by phytoplankton, and some EPS components may have very high affinities for iron (Hassler et al., 2011, 2015; Norman et al., 2015), sometimes at even higher uptake rates than would be expected if all iron were inorganic (Fourquez et al., 2023).
Multiple analytical approaches indicate that a major portion of the marine ligand pool corresponds to highly degraded, refractory organic matter. Numerous studies have detected organic ligands in the ocean with fluorescent and electrochemical characteristics that are similar to reference humic substances composed of recalcitrant organic decay products. These “humic-like” ligands are widespread and often comprise a significant fraction of the organic ligand pools of several metals. Humics incorporate a range of binding sites; while they are primarily considered to be intermediate-strength ligands for iron and copper, some humics (e.g., from the Arctic) may have binding strengths as high as those for siderophores (Laglera et al., 2019).
In the deep ocean, these humic ligands may comprise about 5% of DOC (Laglera and van den Berg, 2009; Dulaquais et al., 2018). In regions that are heavily influenced by terrestrial inputs, such as coastal areas and the Arctic Ocean, iron-humic complexes can be the dominant form of dissolved iron species (Laglera et al., 2019; Slagter et al., 2019). In the Atlantic and Southern Oceans and the Mediterranean Sea, electroactive humics comprise potentially around 20%–60% of the iron-binding ligand pool (Dulaquais et al., 2018; Whitby et al., 2020b; Fourquez et al., 2023). The South Pacific Ocean has the lowest potential contribution of electroactive humics to the ligand pool, ranging from 2% to 51% (Cabanes et al., 2020; Dulaquais et al., 2023). While humic materials have a distinct fluorescent signature, there is a disconnect between distributions of humic-like fluorescent DOM (FDOM) versus electroactive humic-like material (Fourrier et al., 2022), suggesting these fractions measure different components of DOM and that the relationship of these fractions to iron varies regionally. In the North Pacific, humic-like FDOM, dissolved iron, and iron solubility appear correlated (Tani et al., 2003; Yamashita et al., 2020), differing from parts of the Atlantic where this relationship breaks down (Heller et al., 2013; Whitby et al., 2020b). How changes in the contribution of humic material to the total ligand pool might relate to the progressive changes observed in overall iron-binding capacity between the deep waters of the Atlantic and Pacific is currently unclear, but thanks to GEOTRACES, increased spatial resolution is providing new insights into the sources and sinks of metal-binding ligands and their identity at a global scale.
Likewise, analysis of marine ligands by liquid chromatography coupled with inductively coupled plasma mass spectrometry indicates that the majority of solid phase extractable iron, copper, nickel, and likely other elements is present as a complex mixture of molecules that cannot be chromatographically resolved (Boiteau et al., 2016b, 2019). This is consistent with the observation that a major fraction of long-lived DOM is composed of a structurally complex mixture of carboxylic-rich aliphatic small molecules that have been proposed to contain metal-complexing groups (Hertkorn et al., 2013). These refractory organic ligands are likely major buffers of total dissolved metal concentrations throughout the ocean, and thus the magnitude of their sources and sinks are key regulators of global metal distributions. Current evidence suggests that their sources are at benthic boundaries and along coastlines, and that there is a surface sink. Electrochemical measurements indicate the remineralization of sinking particles can also be both a source and sink of humic-like ligands (Whitby et al., 2020a). While their influence on metal bioavailability, particularly in dynamic surface waters, is not yet well understood (Fourquez et al., 2023), the ubiquitous nature of humic-like ligands make them important buffers in supporting the long term oceanic metal inventory (Laglera and van den Berg, 2009; Whitby et al., 2020b).
Overall, GEOTRACES has supported the analysis of a wide range of metal-binding compounds in open ocean waters, such as H2S and polyphenols for iron (Schlosser et al., 2018; González et al., 2019), and thiols (Swarr et al., 2016; Gao and Guéguen, 2018; Whitby et al., 2018), which can bind copper and other metals. There have also been dedicated efforts to understand the role of ligands in iron redox speciation (Santana-Casiano et al., 2010; González et al., 2012; Arreguin et al., 2021; González-Santana et al., 2023). Continued studies are necessary to connect operationally defined ligand classes with specific compounds to better constrain the sources, sinks, and distribution of ligands with distinct chemical properties (Figure 1). Use of key model ligands, such as desferrioxamine B and Suwannee River humic standards, has proven extremely useful in developing our understanding of ocean metal distributions and speciation, but they do not fully represent the entire spectrum of marine ligands. Identifying alternative model ligands, ideally of marine origin, and characterizing the metal binding behavior of marine DOM isolates, will support further advances in this area.
Modeling Metal Speciation and Cycling
Alongside the advances in quantification and characterization of organic ligands, GEOTRACES has sparked advancements in modeling chemical speciation (Turner et al., 2016) and the representation of metal speciation within global ocean biogeochemical models such as PISCES (Tagliabue et al., 2016; Richon and Tagliabue, 2019). Increased data quality and availability, and the development of models such as Marchemspec (http://marchemspec.org/) allow us to assess controls on metal solubility. Progress in these areas has focused on making improvements that can capture measured distributions of trace metals (Tagliabue et al., 2016), with the goal of generating predictive knowledge of how ocean trace metal sources, sinks, and biological impacts will change in the future.
The parametrization of organic ligands has major effects on model outputs of metal distributions and remains a major source of uncertainty. The earliest models proposed that organic complexation extends the residence time of iron in deep waters and that there is a “threshold” strong ligand concentration of 0.6 nM that is uniform throughout the ocean, with no inter-basin differences in deep ocean dissolved iron concentrations (Johnson et al., 1997). Although the “fixed ligand” assumption is still a key feature of many models of global iron distributions, measurements of organic ligand concentrations indicate variations that can exceed an order of magnitude. Some models have incorporated a dynamic ligand pool by tying total ligand concentrations to DOC (Tagliabue and Völker, 2011; Völker and Tagliabue, 2015); more recently, the iron binding capacity of ligands have been parametrized as a function of pH and DOC (Ye et al., 2020). However, expanded measurements comparing DOC and organic ligand concentrations indicate that they have distinct distributions (Figure 1). Where the DOM and ligand pools are largely refractory, such as in deeper, older waters, ligands likely comprise a relatively consistent fraction of DOM. Yet, in productive sunlit waters and at remineralization depths, the production and degradation of more labile ligands results in greater variability in the relative concentrations of ligands and bulk DOC (Hassler et al., 2020). By studying the distributions of specific ligands alongside measurements of the average bulk ligand pool, we are uncovering the sources and sinks of compositionally distinct ligand components that cycle differently. Understanding the environmental factors that control the distributions of these components is crucial for accurately incorporating organic ligands into biogeochemical models.
In addition to contemplation of a dynamic ligand reservoir, there have been advancements in ways to model metal speciation. New speciation models have included NICA-Donnan parameterizations of organic-metal binding that describe complexation of cations to terrestrial humic-like organic matter, and considered a distribution of ligands with different binding strengths. Such models provide an estimation of metal speciation (Hiemstra and van Riemsdijk, 2006; Stockdale et al., 2011) linking DOM bioavailability with iron solubility (Zhu et al., 2021a). These models may be appropriate in the marine setting given recognition that much of the complexation capacity in the ocean may be attributed to polydisperse degradation products and, in accounting for the complexity of seawater DOM, the NICA-Donnan model approach may better predict iron bioavailability in natural waters (Zhu et al., 2021a; Gledhill et al., 2022a, 2022b). However, there are still open questions regarding the extent to which speciation is well described by assumptions of thermodynamic equilibrium and the extent to which different metals may compete for the same metal binding sites.
Very recent work suggests that processes other than organic complexation may exert important controls over the distribution, solubility, and bioavailability of metals in the ocean. Throughout the ocean, a large portion of metals such as dissolved iron exist in “colloidal” phases that are known to be inert to competitive ligand exchange, implying strong stability or slow kinetics of metal binding. Studies have found that the colloidal phase is important for iron (Kunde et al., 2019; Homoky et al., 2021), but less so for some other metals commonly complexed by ligands, such as cobalt, copper, and manganese (Jensen et al., 2021). Colloidal iron makes up a large fraction (up to 85%) of dissolved iron in surface waters of high-dust areas like the tropical and subtropical North Atlantic waters (Fitzsimmons and Boyle, 2014; Fitzsimmons et al., 2015a; Kunde et al., 2019) but comprises a much lower fraction in low-dust areas such as the Southern Ocean and the western Arctic (Boye et al., 2010; Jensen et al., 2020). Despite its abundance in surface waters, colloidal iron sharply declines in the subsurface/deep chlorophyll maximum, which suggests that it may be rapidly scavenged onto particles or taken up by microbes. Recent studies also suggest that colloids of authigenic iron oxyhydroxide phases can form and coprecipitate with organic matter (Curti et al., 2021), which may “shunt” metals such as iron into particles. Including these reactions in a global-scale biogeochemical model resulted in much greater agreement between model simulations and observations (Tagliabue et al., 2023). Work around hydrothermal vents has revealed the importance of the iron redox state (Toner et al., 2009a, 2009b), and shown how humic-like ligands can only solubilize a very small fraction (~1%–5.5%) of aged iron oxyhydroxides (Dulaquais et al., 2023). Complex phases that diverge from the commonly considered 1:1 metal-ligand binding behavior have largely been ignored in the past, illustrating how we may need to consider alternative relationships between DOM and iron cycling, and to reconsider assumptions based on operational definitions surrounding size class and metal speciation.
Moving Forward: Understanding Bioavailability
The chemical speciation of metals strongly influences their bioavailability, which is defined and discussed in the following section based on their phytoplankton uptake kinetics. The free metal ion, M', including the inorganic complexes of M' with Cl–, CO32–, etc., is compatible with transport systems found in all cells. M' is considered as the preferred substate for uptake by phytoplankton, making it the most bioavailable and/or toxic physicochemical form of trace metals (Sunda, 1975; Hudson and Morel, 1990). Laboratory experiments with synthetic organic ligands, such as ethylenediamine-tetraacetic acid (EDTA) or nitrilotriacetic acid (NTA) demonstrated that metal complexation markedly decreased bioavailability and rates of uptake (Hudson and Morel, 1990; Sunda and Huntsman, 2000). The complexation-driven drop in bioavailability results from a decrease in the free metal ion concentration in the ambient medium and the fact that these synthetic ligands are unassimilable (Shaked et al., 2005; Sunda, 2012).
In seawater containing diverse natural ligands, the influence of complexation on uptake kinetics is less clear than it is for laboratory experiments. Some metal complexes such as thiosulfate, low-molecular-weight organic metabolites, and low-affinity ligands can be indirectly utilized or directly taken up by phytoplankton to satisfy their nutritional needs (J.M. Kim et al., 2015; Liu and Campbell, 2017), while others may be unavailable for biological uptake. Metal acquisition rates also vary substantially among organisms due to the diversity of both biological uptake pathways and metal species (Guo et al., 2010; Aristilde et al., 2012; Shaked and Lis, 2012). Interactions among different ligand types can also impact dissociation kinetics and ligand exchange (Sukekava et al., 2024) and thus metal bioavailability. Although an all-encompassing simple link between metal speciation and bioavailability cannot be drawn (Shaked and Lis, 2012; Sunda, 2012), the examples given above indicate how knowledge of metal uptake pathways and rates for specific organisms and chemical forms enables useful predictions of where and how metal stress impacts marine communities beyond what is obtainable by metal concentration measurements alone.
Understanding how environmental factors alter metal speciation is also critical for assessing metal bioavailability and uptake rates. Sunlight and reactive oxygen species can dissociate metals from organic complexes through redox mechanisms or by degrading the organic ligand. For example, photodegradation and release of bioavailable iron from upwelled refractory DOM can induce phytoplankton blooms (Hassler et al., 2020). In addition to DOM, organically complexed iron from different ocean basins was shown to undergo photochemical alterations that increased its bioavailability (Mellett et al., 2018; Shaked et al., 2020). Recent evaluation of in situ iron bioavailability using a large single-cell dataset further suggested that sunlight elevates iron bioavailability in the ocean surface (Shaked et al., 2021). These processes likely render metals that are highly complexed by organic ligands more bioavailable at the surface and attenuate bioavailability with depth.
Changes in pH also affect metal speciation and consequently metal bioavailability. Acidification has been shown to decrease the uptake of iron by marine phytoplankton in a manner consistent with predicted changes in iron speciation (Shi et al., 2010). In other cases, decreasing pH has been shown to either increase or decrease metal bioavailability, depending on the composition of complexing ligands, while high pH can lead to decreased inorganic copper or increased inorganic iron concentrations (Avendaño et al., 2016; Liu et al., 2020). DOM or colloidal aggregates can also influence metal speciation by adsorbing and transporting metals (Aiken et al., 2011; Bressac and Guieu, 2013). Changes in physicochemical conditions, such as temperature and pH, can also impact the abundance and composition of DOM and its metal binding functional groups (Piontek et al., 2009; Zhu et al., 2021b). Such studies provide an important foundation for understanding how metal stress will be exacerbated or alleviated under changing ocean conditions.
It is also important to note that the metal speciation we measure in the laboratory at room temperature and with buffered pH may not best represent in situ speciation. For example, sea ice and brine samples come from a wide range of temperature and salinity conditions, and speciation measurements made under typical conditions can be different from those in the natural environment (Hassler et al., 2013; Genovese et al., 2022). Microscale processes that are not reflected by bulk phycosphere (the micrometer scale region immediately surrounding a phytoplankton cell), such as pH and oxygen concentrations, can also be very different from bulk seawater on which we perform our analyses, resulting in different organic and inorganic speciation (Liu et al., 2020). Cutting-edge research using nanoprobes to directly look at chemical conditions and metal speciation within the phycosphere could help us better understand metal bioavailability in aggregates or colonies that are hotspots of biological activity (Basu et al., 2019; Eichner et al., 2019; Liu et al., 2022). Considering that trace metal bioavailability depends on an interplay of chemical, physical, and ecological factors in the ocean, quantifying metal speciation has the important aim of providing predictive knowledge about which biological processes are influenced by metal stress across the ocean and how they will respond to environmental change.
Conclusions
The GEOTRACES program has collected an abundance of data to help us understand and model the cycling of important trace metals in our ocean, in particular dissolved iron. Speciation studies have allowed us to better explain key processes driving these biogeochemical cycles and to identify some of the major contributors to the ligand pool and their sources and sinks. It is also important to note that GEOTRACES has supported a wide range of highly relevant measurements on metals that extend beyond this article but that have advanced our understanding of ocean metal chemistry. Advances gained through GEOTRACES data will continue to aid us as we investigate the roles of metals in the carbon cycle and the climate, and how, in turn, changes in oceanic conditions due to climate change affect metal speciation. This includes understanding how shifts in pH, temperature, and oxygen levels impact the solubility and speciation of metals in seawater. The continued work to standardize existing procedures along with the development of novel techniques is broadening our understanding of metal behavior in ocean waters while simultaneously presenting many new questions to answer.
We currently lack knowledge of the abundance of specific compounds and of how to directly connect widespread electrochemical speciation measurements with methods that characterize individual compounds within the ligand pool, or with metal bioavailability, including the importance of thermodynamic versus kinetic controls on rates of uptake by phytoplankton. We also lack information on how fast these different compounds cycle and how heterogeneous or regionally specific they may be. No metal exists in isolation; the dynamic environment of sources, sinks, and cycling processes means that metals are in constant competition for binding sites, and they continuously undergo processes that impact their solubility and bioavailability. Future modeling of metal cycling may be improved by understanding the relationship of metal-binding ligands to DOM and the processes that control their production and degradation, as well as expanding our view of metal speciation to include inorganic forms. However, as processes relevant for ligands are not always necessarily directly relatable to those acting on the overall DOM pool, particularly in dynamic upper waters, consideration of the differences in residence times, reactivity, and bioavailability of specific metal-binding ligands will be essential. Ultimately, we still have a long way to go to understand metal speciation and its links to bioavailability, biogeochemical cycling, and the ocean metal inventory, but exciting progress has been—and continues to be—made, spurred by the GEOTRACES era.
Acknowledgments
This research was supported by grant no. 2020041 awarded to YS and RMBoiteau from the United States-Israel Binational Science Foundation (BSF). RMBoiteau also acknowledges US National Science Foundation (NSF) grants OCE-2422713 and OCE-2422713. KNB acknowledges support from NSF grants OCE-2300915 and OCE-2310573. RMBundy was supported by NSF grant OCE-2219626. The international GEOTRACES program is possible in part thanks to the support from the US National Science Foundation (Grant OCE-2140395) to the Scientific Committee on Oceanic Research (SCOR).