The Northwest Atlantic
Circulation in the Northwest Atlantic is dominated by the Gulf Stream, a subtropical western boundary current whose warm, salty waters course along the continental slope of the southeastern United States in a narrow (~100 km width), intense jet that serves both to close the wind-driven gyre and to carry the warm limb of the Atlantic Meridional Overturning Circulation poleward. After passing Cape Hatteras, North Carolina, the deep-reaching Gulf Stream begins to meander, serving both as a moving boundary between the water masses, ecosystems, and chemical regimes of the Slope and Sargasso Seas (Figure 1a) and as a locus of air-sea exchange that drives intense regional wintertime cooling (Joyce et al., 2013) and uptake of atmospheric carbon dioxide (Nickford et al., 2024).
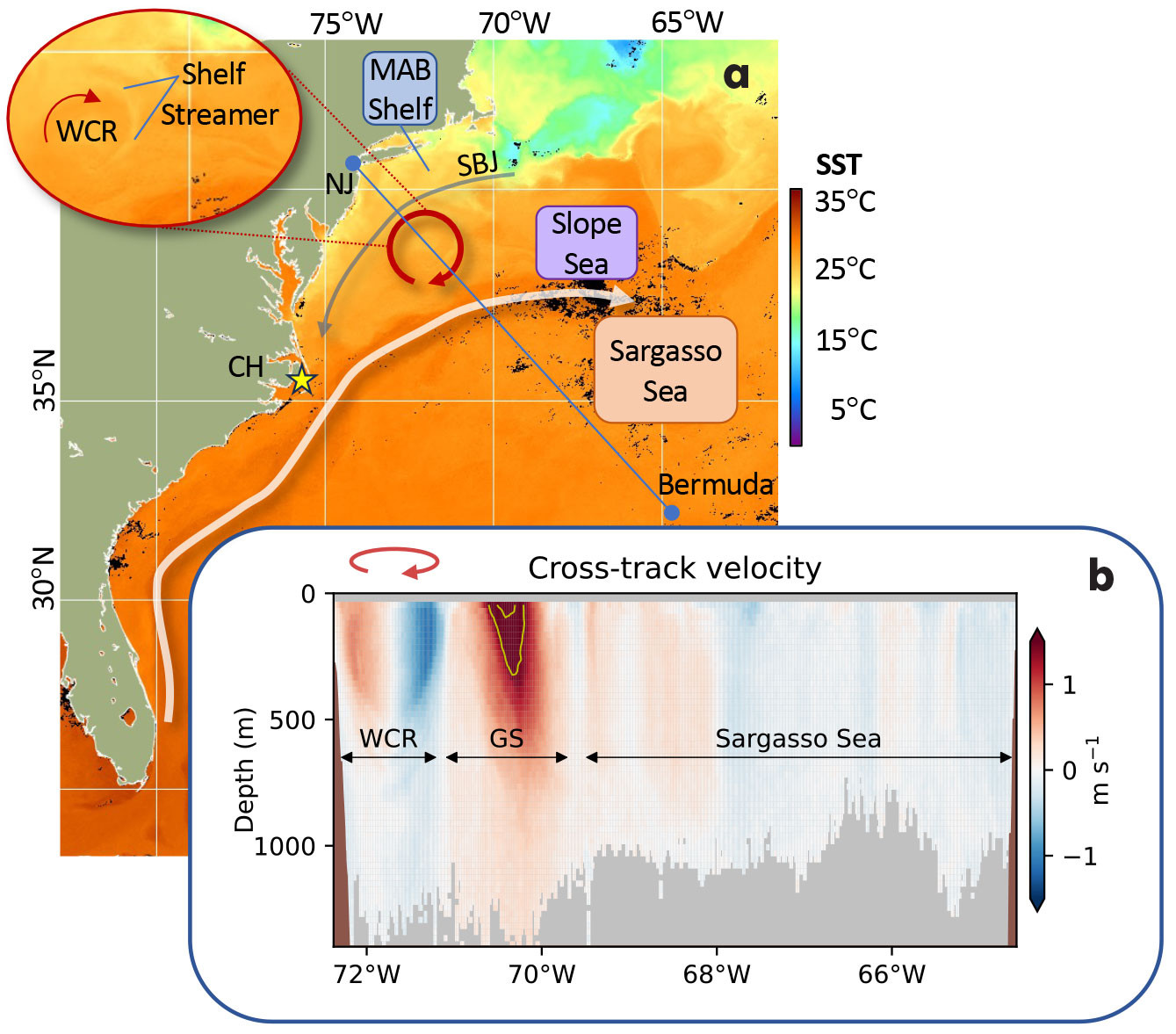
FIGURE 1. (a) Schematic of Northwest Atlantic circulation superimposed on a composite sea surface temperature (SST) map produced by NOAA from the Advanced Very High Resolution Radiometer and the Visible Infrared Imaging Radiometer Suite (AVHRRR/VIIRS) merged from July 23–26, 2021. The inset shows a close-up of a warm core ring (WCR) with a streamer of cooler (and fresher) waters from the Middle Atlantic Bight (MAB) shelf wrapping around the anticyclonic ring. (b) Cross-track component of ocean velocity (heading 47°) measured during the concurrent Bermuda-bound CMV Oleander transit with an Ocean Surveyor 38 kHz ADCP (OS38), with the 1.5 m s–1 and 2.0 m s–1 isolines (yellow) and bathymetry (shaded brown). Gray shaded regions have less than 30% good data returns. The WCR is deep reaching and surface intensified: ~16 Sv is being circulated within the upper 1,000 m of the ring, and most of this (88%) is concentrated in the upper 500 m. The Gulf Stream is carrying ~81.6 Sv (integrated from the surface to 1,000 m depth and from 71.1°W to 69.6°W). > High res figure
|
Warm and cold core rings are intermittently shed from Gulf Stream meander crests and troughs, respectively, and drive transport across the sharp front that separates the deep thermocline (~800 m depth) and salty, warm oligotrophic Sargasso Sea waters to the south from the shallow thermocline (~200 m depth) and relatively cooler, fresher Slope Sea waters to the north. Rings influence biological (Hare et al., 2002) and chemical (Conway et al., 2018) distributions as well as air-sea fluxes (Silver et al., 2021).
Warm core rings, which carry Sargasso Sea waters into the Slope Sea, are deep-reaching and cannot move directly onto the shallow Middle Atlantic Bight (MAB) shelf (Figure 1b). They do, however, interact with the upper slope and outer shelf through ageostrophic processes that impact the Shelfbreak Jet (Forsyth et al., 2022) and that exchange waters across the shelf break (e.g., Zhang and Gawarkiewicz, 2015; Gawarkiewicz et al., 2022). The Gulf Stream also sheds warm filaments called “shingles” (von Arx et al., 1955) into the Slope Sea. The formation, evolution, and impacts of individual shingles, rings, the Shelfbreak Jet, and other (sub)mesoscale circulation features can be difficult to capture with the limited temporal and spatial resolution of satellite observations and intermittent cloud cover. Underway in situ measurements from ships can complement satellites with their high along-track resolution and critical subsurface measurements.
Since the 1970s, scientific equipment has been hosted on three different container ships operating consecutively on the “Oleander Line,” a longstanding route between Elizabeth, New Jersey, and Hamilton, Bermuda. The operation on the first two vessels and key results from 25 years of velocity and 40 years of temperature measurements are summarized in Rossby et al. (2019), with the historical data (see The Oleander Project website) and derived products (Forsyth et al., 2020a, 2020b) available.
Scientific Sensors on the New CMV Oleander
The newest CMV Oleander came into service in 2019 with some scientific infrastructure and sensors already installed, and additional equipment was installed and commissioned upon the ship’s first drydock in early 2024. The vessel is now providing water column, sea surface, and atmospheric measurements along the Oleander Line (Figure 2).
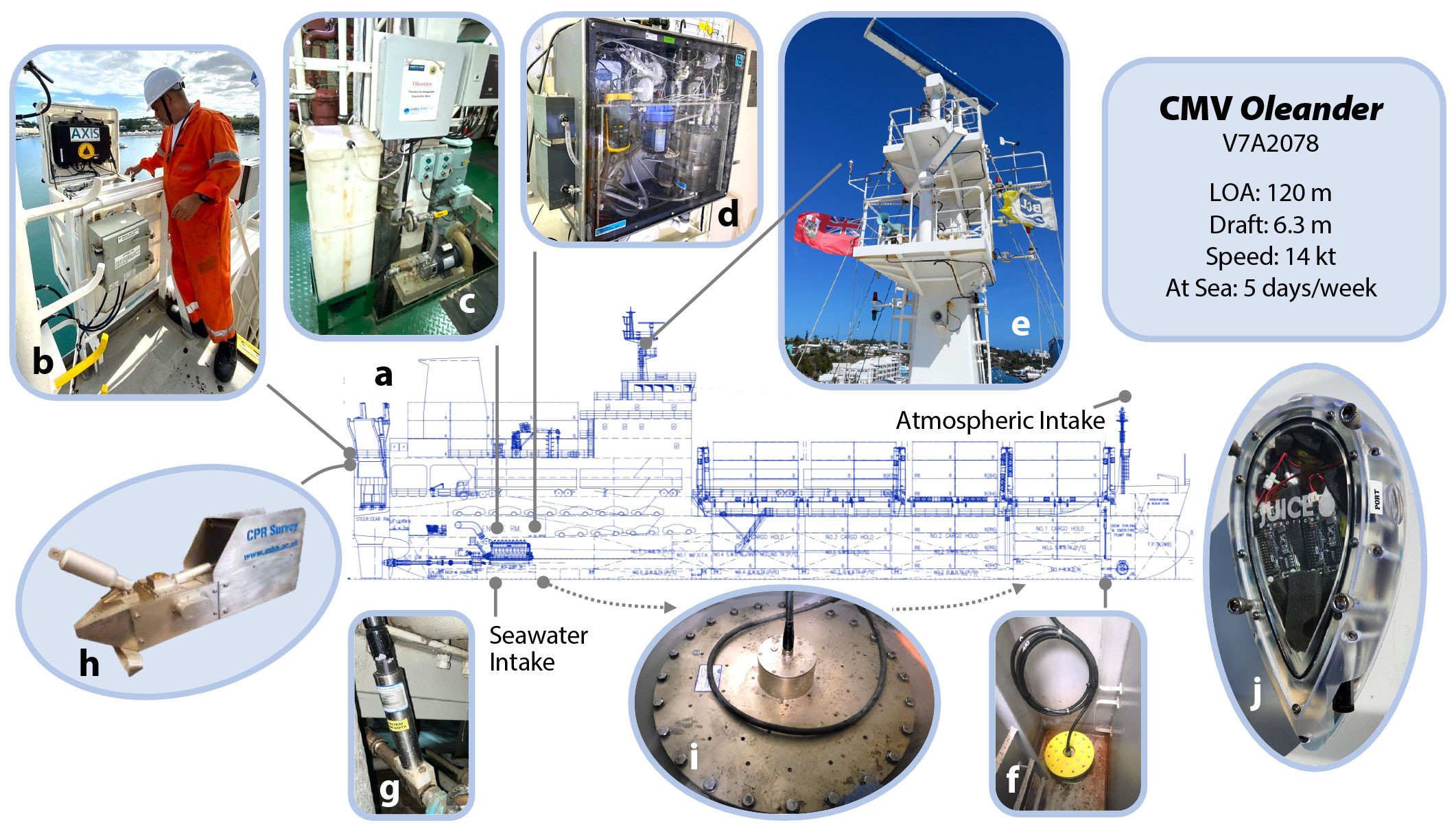
FIGURE 2. (a) General arrangement drawing of the new CMV Oleander showing ship configuration and oceanographic sensors. (b) The Autonomous eXpendable Instrument System (AXIS) on the stern being reloaded with Global eXpendable BathyThermograph (XBT) probes. (c) Underway scientific seawater system in the engine room. (d) Sea-Bird SBE45 TSG for recording seawater salinity (left) and General Oceanics 8050 pCO2 Measuring System (right), with a LICOR 7000 analyzer calibrated every two to three hours with four standard gases with concentrations ranging from 0 ppm to 470 ppm traceable to the World Meteorological Organization (WMO) scale. (e) Vaisala WXT536 weather sensor mounted on the flybridge mast to measure air temperature, humidity, sea level pressure, rainfall, and wind speed and direction. (f) Hull-mounted Teledyne RD Instruments 150 kHz Ocean Surveyor ADCP (OS150) with a 3/8-inch-thick polycarbonate acoustic window for protection. (g) Sea-Bird SBE38 temperature probe near the seawater intake to provide near-surface ocean temperature before the seawater pipes pass through the hot engine room. (h) Continuous Plankton Recorder (CPR), which is lowered by the crew using the ship’s mooring winch for towing behind the vessel. Not shown are the GPS and ABXTWO antennas used for accurate ship position and heading to calculate ocean velocities. (i) The Ocean Surveyor 38 kHz ADCP (OS38), installed during the ship build, has only rarely given good velocity sections, with some profiles reaching beyond 1,000 m depth (e.g., Figure 1b). The OS38 was removed during the January 2024 drydock and will be reinstalled in a forward location with less bubble noise and with a 1½ inch acoustic window for protection. The new site was chosen based on analysis of videos of the bubble field using (j) the commercially available, hull-mounted Remora system with programmable forward- and side-facing cameras built by Juice Robotics LLC. This magnetically mounted camera system was installed by commercial divers for two transits in fall 2023 to help identify the locations of bubble clouds entrained under the vessel that can cause noise in ADCP measurements. > High res figure
|
Measurements include discrete profiles of upper ocean temperatures along the transect. Since late 2020, 48 eXpendable BathyThermograph (XBT) probes have been launched monthly using an Autonomous eXpendable Instrument System (AXIS; Fratantoni et al., 2017). Near-real-time profiles are transmitted via the Iridium satellite network, and after quality control, complete temperature sections are posted on the project’s ERRDAP server and on the XBT project’s website.
Velocity profiles and acoustic backscatter intensity to ~200 m depth are continuously recorded during each crossing with a 150 kHz acoustic Doppler current profiler (ADCP). Individual pings are five-minute averaged to give profiles with along-track resolution of about 2 km. A sample of the ADCP data is sent to shore daily via Starlink to monitor system performance, and the full dataset is downloaded during each port call. Velocity sections have been collected biweekly since October 2023 and are available here.
CMV Oleander is equipped with an underway scientific seawater line. The intake, located at ~5.8 m depth, supplies flow-through instrumentation that measures near-surface temperature and salinity. There is a newly installed (since 2024) carbon dioxide (CO2) system for measuring partial pressure and fugacity of CO2 (pCO2 and fCO2) in surface seawater and boundary layer air, with other sensors occasionally measuring alkalinity and pH. In addition, underway near-surface atmospheric data are recorded with a weather station. The underway data are recorded at 1–4 Hz (except for pCO2, which is recorded every two minutes) and are transmitted to shore via Starlink at 10-minute intervals.
Each month, the ship tows a Continuous Plankton Recorder (CPR) at ~10 m depth to sample plankton in the upper water column with a 280-micron mesh gauze. CPR cartridges are returned to shore after three or four months and are analyzed to identify and count organism taxa and, where possible, species. The gauze is cut into slices representing ~10 nautical miles of tow, with each slice sampling roughly 3 m3 of seawater. To avoid potential interference between the XBT probes and the towed body, the monthly CPR and XBT sections are generally conducted during separate crossings.
Two recently funded pilot programs will expand the underway biological sampling. A biomolecular autosampler, the Robotic Cartridge Sampling Instrument (RoCSI), and an Imaging FlowCytobot (IFCB) will be connected to the scientific seawater line in 2025. The autosampler will preserve water samples for environmental DNA metabarcoding to assess biodiversity and monitor ecosystems across marine gradients (Adams et al., 2023). The IFCB will provide size- and taxon-resolved concentrations of phytoplankton and their biomasses (Sosik and Olsen, 2007).
Using Oleander Data to Study Processes Across Spatial and Temporal Scales
Along-Track Variability
Concurrent measurements from CMV Oleander’s scientific sensors underscore the interplay of ocean physics, chemistry, and biology at temporal scales spanning events to seasons and spatial scales spanning shingles to regional recirculation cells. For example, velocity profiles recorded during the transit on August 3–4, 2024, provide context for the along-track property distributions (Figure 3). The vessel crossed a ~200 m thick Gulf Stream shingle in the Slope Sea and a deep-reaching cyclonic cold core ring (CCR) in the Sargasso Sea. In this late summer section, heating has muted near-surface temperature contrasts, so the shingle is only slightly warmer than the ambient Slope Sea waters, and the CCR does not stand out as particularly cold relative to the Sargasso Sea. However, near-surface salinities combined with the subsurface temperature profiles (with the 12°C isotherm used to identify the depth of the thermocline) clearly delineate fronts that align with the submesoscale (shingle) and mesoscale (CCR and Gulf Stream) circulation features.
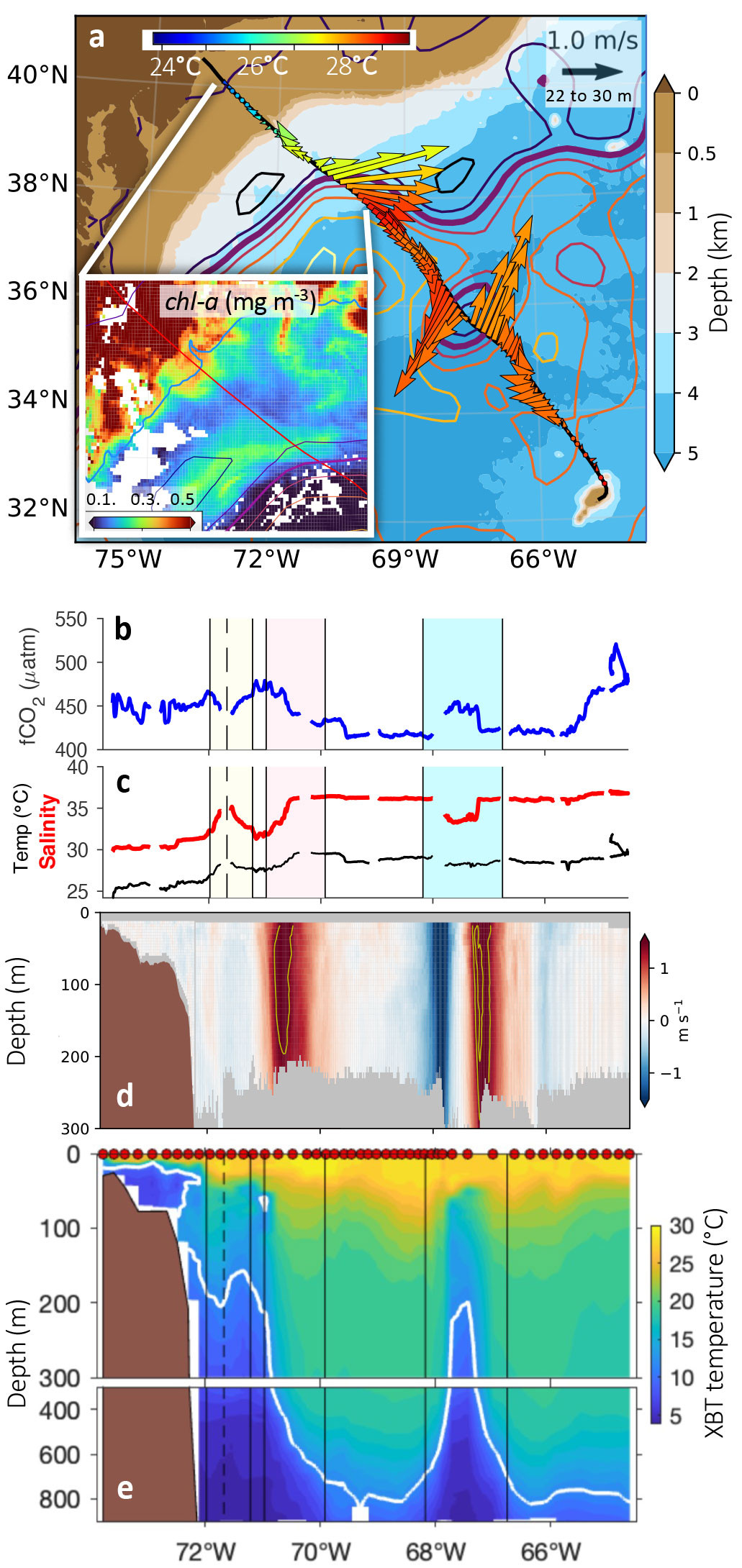
FIGURE 3. Observations spanning August 3–4, 2024. (a) Near-surface velocity vectors (averaged from 22 m to 30 m depth) from the Teledyne RD Instruments 150 kHz Ocean Surveyor ADCP (OS150). Colors, superimposed on bathymetry (shading), indicate temperature as measured internally. The inset shows chl-a from NOAA’s Sentinel-3A-OLCI for August 2, 2024, with the CMV Oleander route plotted in red and the 200 m isobath in blue. Sea surface height contours for August 3, 2024, are also plotted based on mapped satellite altimetry from Copernicus Marine Service product SEALEVEL_GLO_PHY_L4_NRT_008_046 contoured at 0.25 m intervals, with the 0.25 m contour highlighted as a proxy for the Gulf Stream core (thick purple). (b) Underway fCO2 with the shingle (yellow), Gulf Stream (pink), and cold core ring (blue) highlighted. (c) Underway near-surface salinity (thick, red) and temperature (thin, black) with shading as in (b). (d) Cross-track velocity profiles (heading 47°) with the 1.5 m s–1 and 2.0 m s–1 isolines (yellow) highlighted. (e) Temperature section in the upper (surface to 300 m depth) and mid-depth (300–900 m) water column, as observed with XBT probes launched by AXIS at the locations indicated by red dots at the surface, with the 12°C (white) and boundaries of the circulation features noted above (vertical lines). > High res figure
|
The correspondence between these circulation features and the along-track variability in fCO2 is striking. The core of the fresh, cyclonic CCR stands out as a region of elevated fCO2 (450 μatm) within the otherwise lower fCO2 waters of the surrounding Sargasso Sea (i.e., the oligotrophic subtropical gyre of the North Atlantic Ocean). The salty shingle in the Slope Sea, which grows westward as it continues to draw waters from the eastward-flowing Gulf Stream, has lower fCO2 (440 μatm) and, like the Gulf Stream, is more saline than the ambient Slope Sea. The addition of other CO2 sensors will clarify whether the CCR is similar to Slope Sea waters, with higher fCO2 resulting from a higher dissolved inorganic carbon (DIC) to alkalinity ratio, and potentially the contribution of vertical mixing upward of DIC water into the CCR. Concurrent satellite ocean color measurements (see inset to Figure 3a) of chlorophyll a (chl-a) show that—in contrast to the core of the Gulf Stream, which has low chl-a (~0.1 mg m–3)—the shingle is associated with a filament of elevated chl-a (~0.3 mg m–3), suggesting biophysical interactions rather than simple horizontal advection of Gulf Stream water masses by the shingle. Because these features are long-lived compared to Oleander sampling intervals, it will be possible to examine the plankton distributions within these features as sampled by the CPR, which was towed on the previous Bermuda-bound transit on July 27–28, 2024, once the cartridge is returned to shore. The concurrent underway data (near-surface salinity and velocity profiles) will help identify the exact locations of the fronts and circulation features during that transect (which marked the 535th CPR tow from an Oleander).
The (sub)mesoscale variability of fCO2 in the along-track data is superimposed on strong regional-scale contrasts between waters of the coastal Middle Atlantic Bight (~450 μatm), the Slope Sea (~470 μatm), the Sargasso Sea (~420 μatm), and those north of Bermuda (~430–470 μatm) with the waters on the Bermuda reef and lagoon (>490 μatm). While it is known that the assemblages of plankton species in the Sargasso Sea and Slope Sea vary, and that rings can host species not found in surrounding waters, a thorough comparison of the circulation features with the plankton survey and with underway near-surface data (including fCO2) in this and other sections remains to be undertaken.
Long-Term Changes
The observations along the Oleander Line resolve seasonal and interannual variability superimposed on long-term changes on the Middle Atlantic Bight shelf and within the Shelfbreak Jet (Forsyth et al., 2015). Here we show that the measurements also capture significant changes in the open ocean, including warming and low frequency shifts in Gulf Stream position. These changes are in contrast to Gulf Stream transport, which is relatively stable at the Oleander Line (e.g., Rossby et al., 2019), consistent with sustained observations of this western boundary current 1,500 km upstream in the Florida Straits (Volkov et al., 2024).
In the Slope Sea, warming from the surface to 750 m depth spanning the last 86 years (1937–2023) is evident from temperature profiles along the Oleander Line between 39.2°N and 38.4°N, averaged over three different epochs (Figure 4a). Profiles that sampled a warm core ring or a Gulf Stream meander crest can be identified by the shape of the temperature profile and are excluded from the averaging to isolate changes within ambient Slope Sea waters. The mean temperature profiles demonstrate significant, surface-intensified warming of the Slope Sea that is consistent with other studies and is concentrated in the last three decades. Because the number of warm core rings shed annually by the Gulf Stream doubled after 2000 (Gangopadhyay et al., 2019), this upper-ocean warming likely reflects the cumulative effect of mixing of Gulf Stream and Sargasso Sea waters into the ambient Slope Sea by dissipation of warm core rings, which, as noted earlier (Figure 1b), extend to ~750 m depth.
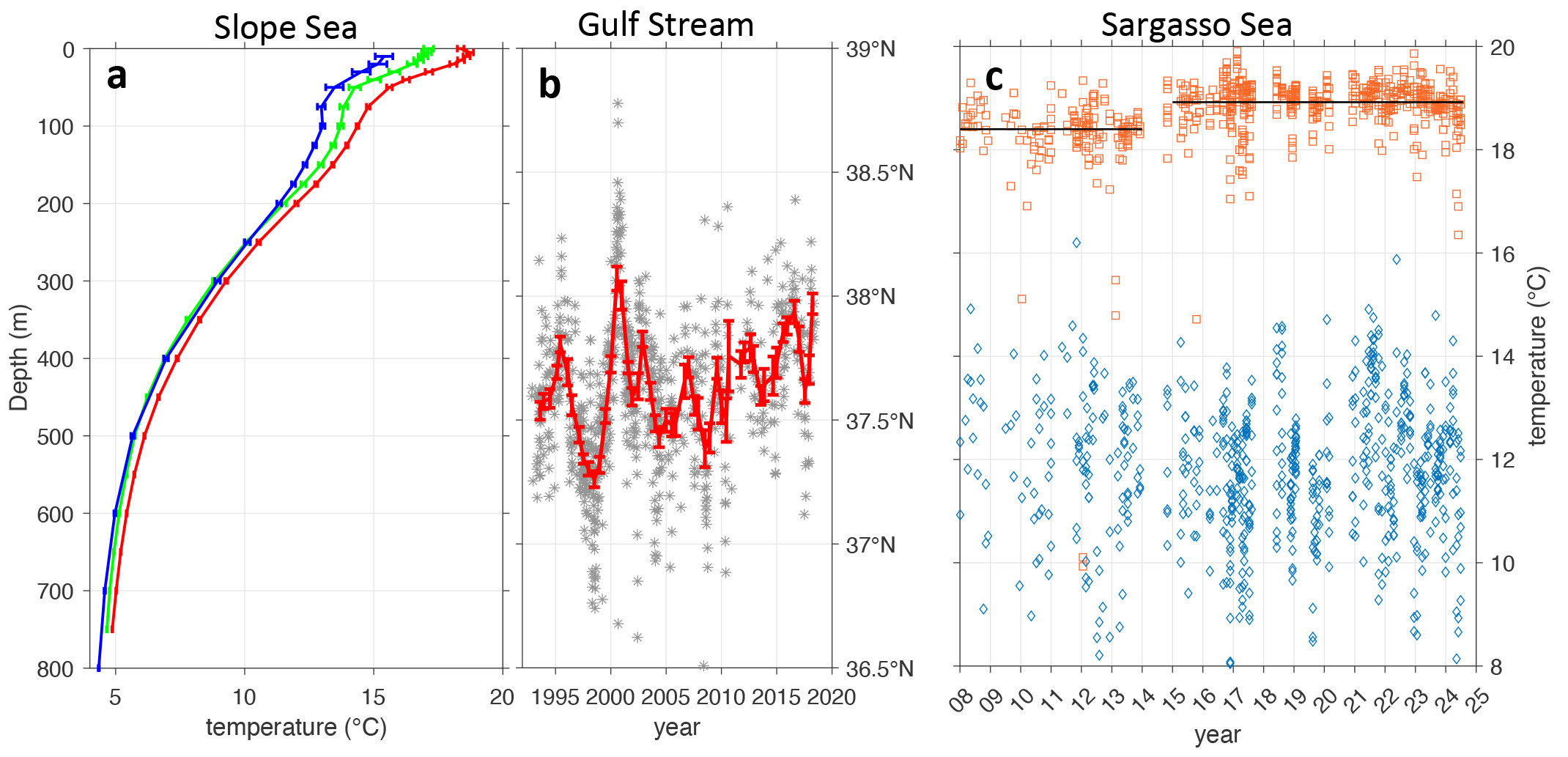
FIGURE 4. Long-term changes in the Northwest Atlantic. (a) Deseasoned and spatially averaged temperature profiles and mean standard errors (horizontal bars) from the Slope Sea for 1937–1940, (blue) from 35 hydrocasts (Iselin, 1940), (green) from 147 XBT profiles collected from 1994 to 2003, and (red) from 284 XBT profiles made during 2014–2023. (b) Latitude of maximum velocity of the meandering Gulf Stream as measured during individual transits (gray stars), with the annual average stepped every six months superimposed (red). (c) Temperature at 300 m depth (red squares) and 800 m depth (blue diamonds) from XBT profiles taken within the Sargasso Sea. Horizontal black lines show time averages at 300 m depth. > High res figure
|
In concert with this warming, the Slope Sea is also shrinking. Oleander velocity sections demonstrate that its southern boundary, the Gulf Stream, has shifted northward by about 50 km (0.5° latitude) since the early 1990s (Figure 4b), with its position identified in each ADCP transect by the latitude of the velocity maximum at 55 m depth. This gradual northward shift and the strong lateral position changes evident in the 1990s and early 2000s may reflect variations in dense water formation and export from the Labrador Sea (e.g., Bisagni et al., 2017).
Temperature profiles from the Sargasso Sea along the Oleander Line between 33°N and 35°N show warming at 300 m depth, with a rather sudden 0.5ºC increase in the mean around 2015 (red, Figure 4c). Warming at this depth reflects change in the subtropical mode water, which used to be called “18°C water” (e.g., Joyce et al., 2013), but which is now 19°C. This change may reflect warmer winters in the mode water formation regions. In contrast, temperatures at 800 m depth show no trend or step change but do show substantial scatter. This scatter, possibly due to internal waves, is not unexpected, as this is the depth of the main thermocline in the Sargasso Sea, and the 1°C standard deviation in temperature here corresponds to about 40 m of thermocline heave.
Global Observing Programs
The Global XBT Network provides repeated upper ocean (0–900 m) temperature measurements along fixed transects at eddy-resolving scales in regions critical for monitoring and understanding upper ocean dynamical and thermodynamic processes. Deployment of XBTs began in the 1960s, and data collected from XBTs became the largest contributor to the upper ocean thermal record during the 1970s–1990s. Since initiation of the Argo array in 1999 to sample the ocean interior, the focus of the XBT network has been to monitor boundary currents, gyre circulation, and meridional transport of heat and mass from trans-basin sections (e.g., Goni et al., 2019). Some XBT transects have been occupied continuously for more than 30 years, providing an unprecedented long-term climate record at spatial and temporal scales that remains unmatched by other observing platforms. XBT profiles have been collected from CMV Oleander (referred to as transect AX32) for nearly 50 years. Starting in 1977, data were mainly collected in the Middle Atlantic Bight shoreward of the Gulf Stream. Since 2009, XBTs have been deployed along the entire section with resolution varying from 15 km within the Gulf Stream to 25–50 km in the ocean interior.
The pCO2 system and associated sensors aboard CMV Oleander allow for evaluation of surface seawater CO2-carbonate chemistry and air-sea CO2 gas exchange over weekly, seasonal, and longer timescales, and across different ocean regions. Such data collection is important for understanding physical and biogeochemical variability at the Bermuda Atlantic Time-series Study (BATS) site (1988 to present; Bates and Johnson, 2023) near the island of Bermuda. The pCO2 data contribute to the international Surface Ocean CO2 Atlas (SOCAT) effort, which provides the scientific community with a global, quality-controlled dataset and gridded product every year (e.g., Bakker et al., 2022). This product in turn contributes to annual global carbon budget analyses (Friedlingstein et al., 2023). It is also the source of multiple other products and publications. The CMV Oleander dataset provides unmatched coverage (100 transects/year) that is uniquely suited to help quality control other regional data sets.
The CPR Survey, established in 1931, seeks to observe the location and abundance of plankton globally. Sampling from ships running southeastward from New Jersey toward Bermuda—referred to as the MB route—began in 1976, with CMV Oleander recruited in 1981 (Jossi et al., 2003). Operations, initially managed by NOAA Fisheries, are now carried out by the Marine Biological Association (e.g., Helaouet et al., 2024).
Future Ocean Observing
The long-standing cooperation between scientists and the Bermuda Container Line/Neptune Group serves as a model for advancing a sustained global observing system that also resolves local and regional processes. Science Research on Commercial Ships (Science RoCS) aims to emulate this enduring partnership on a broad scale (Macdonald et al., 2024), including with the use of ADCPs (Ocean Scope Working Group, 2012), by engaging ship owners and operators. The industry has signaled that it is willing to help as scientists seek to expand their ability to collect sustained observations of the atmosphere and upper ocean waters to advance science and address pressing global challenges. With its integrated system of scientific sensors, CMV Oleander serves as an interdisciplinary observatory in the Northwest Atlantic that can be replicated elsewhere to aid oceanographers who have only limited access to the seas. The successes of Oleander’s operation over the last 50 years demonstrate that partnering with the merchant marine can greatly increase this access.
Acknowledgments
The National Science Foundation (NSF) Division of Ocean Sciences (OCE)-funded Oleander Program supports the operation of the ADCPs, weather station, TSG, and associated infrastructure, as well as the operation of AXIS, with the XBT probes supplied by NOAA/AOML and analysis support from OCE-2122726. Operation of the fCO2 system is funded by NOAA AOML, and the operation of the CPR and analysis of the samples are presently supported by NOAA through the Northeastern Regional Association of Coastal Ocean Observing Systems (NERACOOS). The US Office of Naval Research funded the purchase of a Remora hull-mounted camera system. The expanded biological sampling is supported in part by the Arizona State University/Bermuda Institute of Ocean Sciences Cawthorn Innovation Fund and the Woods Hole Oceanographic Institution Ocean Vital Signs Network Initiative. CMV Oleander science is made possible by the continued generosity of the Bermuda Container Line/Neptune Group and the invaluable expertise and support of the ship’s captains, chief engineers, and crew.