A Short History of Gakkel Ridge Exploration
Forty-five years after the discovery of hydrothermal vents (Corliss et al., 1979), research into these unique habitats and their rich submarine ecosystems has brought about revolutionary findings in biology, chemistry, and geophysics. Understanding how these distinctive ecosystems are supported by sunlight-independent microbial primary productivity based on chemosynthesis changed the way we understand life on Earth (Van Dover et al., 2018). They have inspired our understanding of the origin of life on Earth (Martin et al., 2008) and are now influencing the choice of exploration targets aimed at the discovery of extraterrestrial life in our solar system (Hand and German, 2018; Hand et al., 2020). The exotic faunal communities at active hydrothermal vents are also of high interest given their physiological adaptations and the high degree of endemicity, and for their potential in providing marine genetic resources of use in biomedicine, cosmetics, and biofuels, among others (Van Dover et al., 2018). In addition, interest in the potential for mineral resources in hydrothermal vent deposits has greatly increased in the last two decades, and exploration licenses for such resources have been granted for national and international waters (Jones et al., 2020).
Since the discovery of deep-sea hydrothermal vents in 1977, just over 30% of the global mid-ocean ridge system has been investigated (Beaulieu et al., 2015). To date, exploration has yielded an inventory of 722 confirmed high-temperature vent sites, with a further 720 high-temperature vents inferred from water column data, as reported in the InterRidge Vents Database in September 2022 (Beaulieu and Szafranski, 2020). There may be hundreds of additional active hydrothermal systems and their associated faunal communities yet to be discovered worldwide along the unexplored branches and sections of the global mid-ocean ridge system, particularly along the least explored slow and ultra-slow spreading ridges (Beaulieu et al., 2015).
Current data on vent communities globally has identified 11 biogeographic provinces, but their delineation is still being debated (Rogers et al., 2012). Until now, the vent faunal communities of the ice-covered Gakkel Ridge in the Central Arctic Ocean remained unexplored because of their remote and climatologically challenging location. This study puts the Aurora Vent Field of the Gakkel Ridge on the global map of chemosynthetic-based ecosystems, providing an initial overview of the vent field and the ecosystem it supports.
The Gakkel Ridge (Figure 1a) extends 1,800 km from the northern end of the Lena Trough off Northeast Greenland (81°N) to near the Siberian shelf at 87°N. It was initially predicted to host an extremely low number of active sites based on the assumption that hydrothermal flux scaled directly with spreading rate (E.T. Baker et al., 1996). This hypothesis was revisited after exploration of the Southwest Indian Ridge showed that even ultra-slow spreading ridges could host abundant submarine venting (German et al., 1998). Technological and methodological challenges of working at great depth in regions of permanent sea ice cover have constrained the exploration of the Gakkel Ridge. In 2001, Edmonds et al. (2003) obtained first evidence of hydrothermal venting on nine to twelve discrete locations along the Gakkel Ridge during the InterRidge two-icebreaker (R/V Polarstern and USCGC Healy) Arctic Mid-Ocean Ridge Expedition (AMORE; Figure 1a). Continued exploration during the Arctic Gakkel Vents (AGAVE) expedition in 2007 provided evidence of explosive volcanism at 85°N and demonstrated that large-scale pyroclastic activity is possible along even the deepest portions of the global mid-ocean ridge volcanic system (Sohn et al., 2008). Seismic studies suggest substantial magmatic activity, serpentinization, and fluid flow at this slowest of all Earth’s ridge systems (Michael et al., 2003; Schlindwein and Schmid, 2016). Between 2002 and 2010, the ChEss program aimed to improve understanding of the global biogeography of chemosynthetic-based ecosystems (M.C. Baker et al., 2010). Based on the increasing evidence of hydrothermal venting along the Gakkel Ridge, the ChEss program identified a number of poorly investigated regions where research efforts should focus. The Gakkel Ridge was recognized as one of the missing pieces of the global biogeographic puzzle (Ramirez-Llodra et al., 2007).
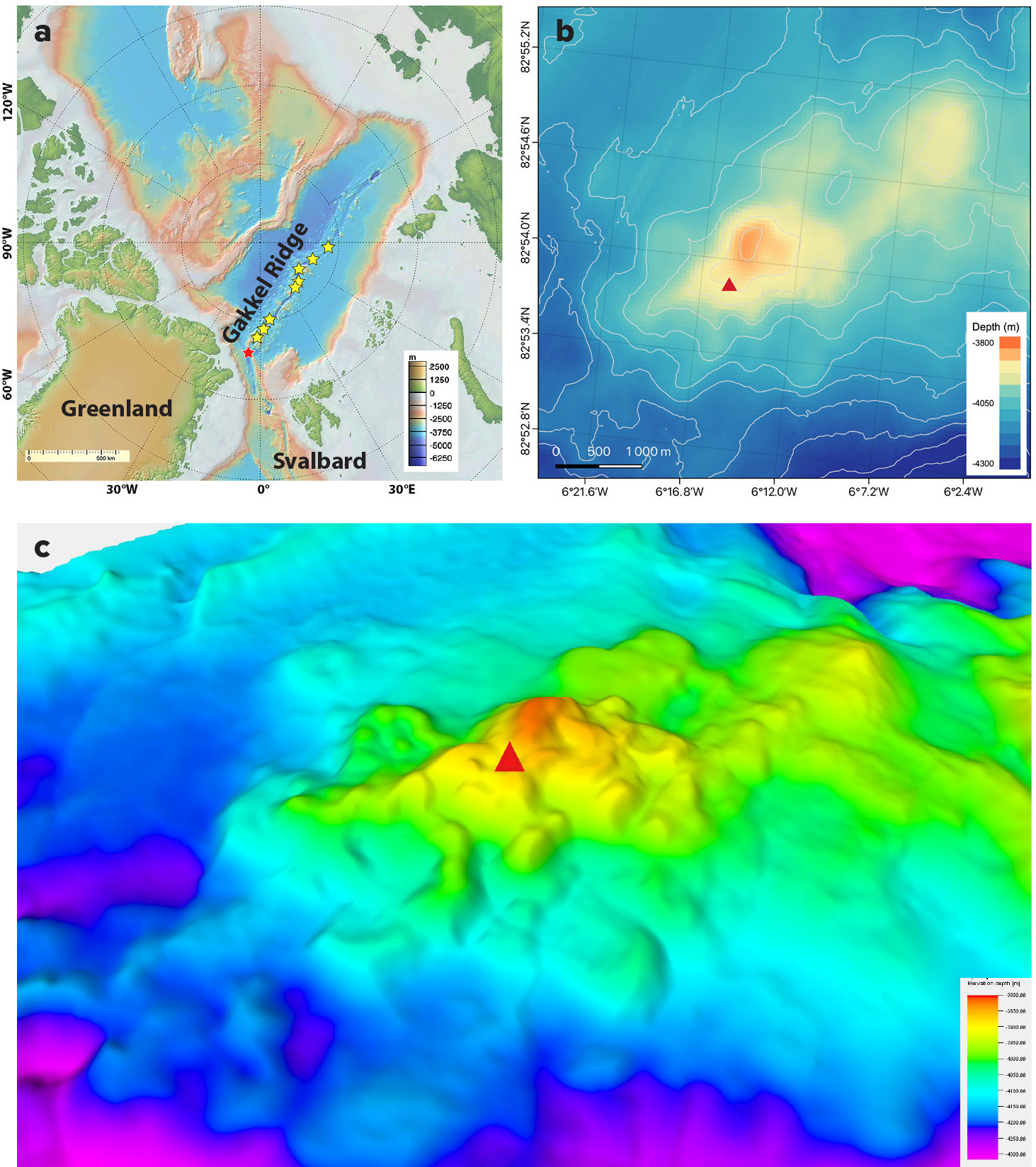
FIGURE 1. (a) Map of the Gakkel Ridge in the Central Arctic Ocean with known hydrothermal plume signals indicated by yellow stars (from Edmond et al., 2003) and the Aurora Vent Field marked with a red star. (b) A red triangle locates the Aurora Vent Field within the Aurora mound based on previous bathymetry from the AMORE and AURORA/AWI expeditions. (c) The Aurora Vent Field (red triangle) is shown against multibeam bathymetry of the Aurora mound based on bathymetry from the HACON19 and HACON21 expeditions. > High res figure
|
Building on the results of the AMORE 2001 expedition (Edmonds et al., 2003), in 2014, R/V Polarstern expedition PS86 AURORA aimed to study geophysical, geological, geochemical, and biological processes at hydrothermal vents on the Gakkel Ridge, with a focus on the southern segment (Boetius, 2015). In this region, the spreading rate is 14.5–13.5 mm yr–1, (slightly faster than the average rate for the overall ridge), and the ridge axis floor at 4,200 m depth is bounded by steep rift valley walls and punctuated by a series of axial volcanic ridges and smaller volcanic mounds (Michael et al., 2003). Edmonds et al. (2003) inferred the presence of an active hydrothermal vent site from chemical data and assigned to a small (1.5–2 km in diameter) volcanic mound rising approximately 400 m from the seafloor, at depths between 4,300 m and 3,850 m (Figure 1b,c). A dredge from south to north across this mound recovered components of a sulfide chimney in addition to abundant pillow basalts. In parallel, in situ sensor data from a MAPR (Miniature Autonomous Plume Recorder) instrument attached to the dredge revealed evidence for a turbidity anomaly consistent with a nearby source of active black smoker venting at a depth of 2,800–3,400 m (Edmonds et al., 2003; Michael et al., 2003). During the PS86 AURORA expedition, CTD profiling, coupled with water column chemistry, revealed further evidence for ongoing hydrothermal activity on the Aurora mound (Boetius, 2015; German et al., 2022a). Seabed surveys with the Ocean Floor Observation System (OFOS) deep-tow camera across the summit from north to south revealed deep rifts through the thick sedimented seafloor across the base of the volcanic mound. This imaging, paired with CTD data, led to the first imaging of an active black smoker on Gakkel Ridge at 82°53.83'N, 6°15.32'W, at ~3,900 m depth, on what was named the Aurora Vent Field (AVF; Boetius, 2015; German et al., 2022a). The OFOS surveys showed that the Aurora mound has steep vertical basalt walls intermixed with lower angle, sediment-draped steps. The top of the mound is flat and sediment covered, and the observed fauna consisted of high abundances of filter feeders, mostly glass sponges and anemones, and at least two species of shrimp. Ophiuroids, swimming polychaetes, and crustaceans (potentially isopods) were also observed. At the active vent site, bacterial mats and small gastropods and amphipods were observed (Boetius, 2015). The physicochemical and microbiological characterization of the huge buoyant vent plume hovering above the AVF showed evidence for venting fluids enriched in methane, and possibly hydrogen, fueling high microbial activity in the plume (German et al., 2022a; Massimiliano Molari, Max Planck Institute for Marine Microbiology, pers. comm., 2022). Due to the lack of a deep-diving remotely operated vehicle (ROV), however, no physical samples of fluids, rocks, microbes, or animals could be collected from the vent field.
In 2019, the Hot Vents in an Ice-Covered Ocean (HACON19) cruise on R/V Kronprins Haakon returned to the Aurora mound, with the aim of conducting a multidisciplinary survey of the seafloor ecosystems centered around the coordinates of the black smoker identified in 2014 by the PS86 Aurora team (Boetius, 2015). This cruise obtained new visual data of the AVF with the towed Ocean Floor Observation and Bathymetry System (OFOBS; Purser et al., 2019; German et al., 2022a), confirming the presence of at least three black smokers colonized by sparse fauna composed of mostly gastropods and amphipods (Bünz et al., 2019). In addition, a wealth of samples on the sedimented surface of the Aurora mound were collected, using coring equipment as well as the Woods Hole Oceanographic Institution hybrid ROV/AUV Nereus Under Ice (NUI), which collected sponges for taxonomic analyses. However, technological challenges and ice conditions prevented NUI from reaching the vent field and collecting samples (Bünz et al., 2019).
Between June and September 2021, the Joint Arctic Scientific Mid-Ocean Ridge Insight Expedition (JASMInE) carried out the first wide-angle reflection/refraction seismic experiment along the Gakkel Ridge, from 75° to 102°, to map lithospheric structure (Ding et al., 2022). Preliminary results contribute to the understanding of oceanic crustal formation and episodic magmatism in this endmember of global oceanic crustal accretion (Ding et al., 2022).
Later in 2021, the HACON21 cruise wrote the latest chapter in the exploration of Gakkel Ridge, contributing to the UN Decade of Ocean Science for Sustainable Development through the Challenger 150 programme (Howell et al., 2020). A multidisciplinary team sailed aboard R/V Kronprins Haakon from Longyearbyen (Svalbard) on September 29 to complete the first ROV survey and sampling of hydrothermal vents under permanent ice cover in the Arctic (Bünz et al., 2021). Below, we describe the methodological approach used to successfully dive on and sample deep hydrothermal vents under drifting ice with an ROV, providing an operational baseline for future robotic explorations of the deep Arctic. The second part of the paper provides a preliminary description of the Aurora Vent Field and its biological communities.
ROV Deep-Sea Exploration Under Ice
Vessel Positioning
The ocean surface above the Aurora mound is permanently covered by sea ice (Figure 2a). The maximum sea ice extent in the Arctic occurs at the end of winter, generally in March, and the minimum extent is normally observed in September, at the end of the summer season, so mid-August to mid-October is the most suitable time window for oceanographic operations on the Gakkel Ridge. Reaching a specific study site in an ice-covered ocean is the first challenge to overcome. Ice floe size, coupled with the speed and direction of ice drift, will determine the time it takes for a specific subsea location to be accessible. The speed and direction of ice drift varies within and between days, driven by tidal forces and wind conditions, creating an ever-changing icescape for which we have limited predictability on the order of 6–12 hours (Boetius, 2015). The presence of thin-ice/open-water leads and pressure ridges between ice floes greatly influences the ability, time, and effort needed to reach the study area.
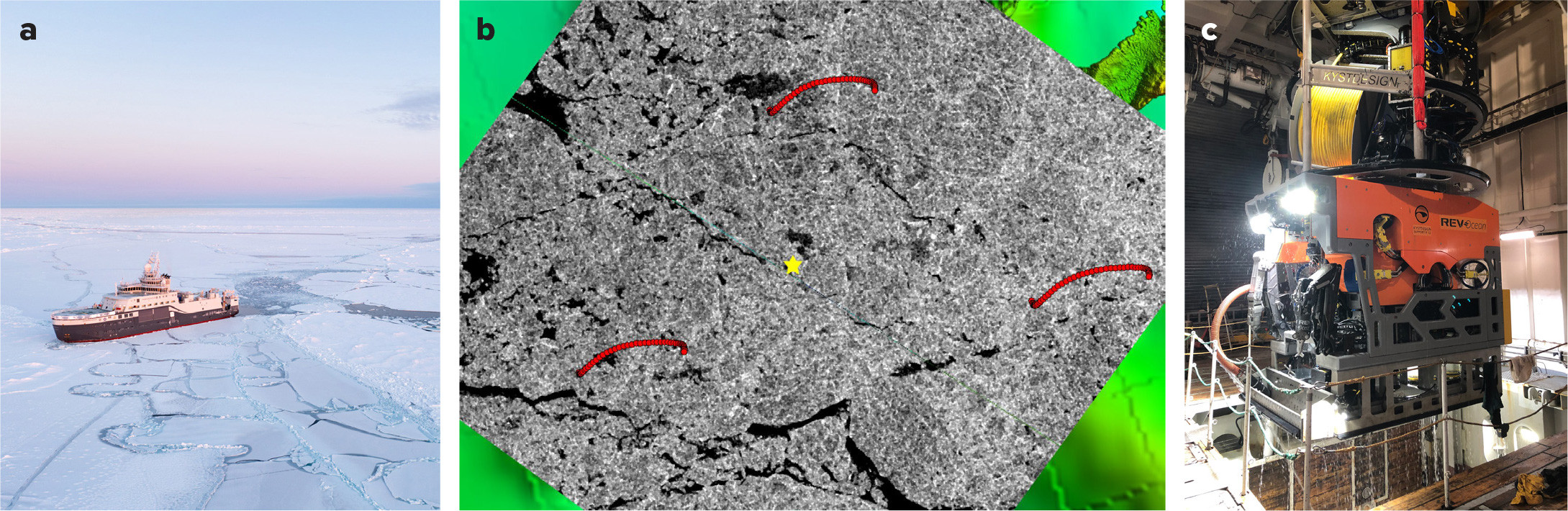
FIGURE 2. (a) R/V Kronprins Haakon navigates a thin-ice lead between ice floes above the Aurora Vent Filed. © REV Ocean/L. Hislop (b) This satellite image shows the regional ice situation and the ice drift predictions (red dots) modeled by Drift+Noise Polar Services GmbH/AWI. The darker areas are open water or thin ice. The gray/white coloring indicates thicker ice. The yellow star indicates the position of the Aurora Vent Field. (c) ROV Aurora Borealis is recovered through the moonpool of R/V Kronprins Haakon along with its 1,000 m tether management system (TMS). > High res figure
|
When the vessel has reached the study area, the first step in planning ROV or OFOBS dives is to determine and predict ice-drift velocity and direction in order to position the vessel within the context of current and developing ice conditions. Once the ROV is launched, the ship will no longer be able to reposition, so it must be located “upstream” of the dive target. The aim is for the ROV to reach the seafloor ahead of the vent field/study site, while the vessel drifts with the ice floe toward the study site. To determine vessel positioning, we used satellite images that provided a regional overview of sea ice conditions (i.e., ice floe sizes, their distribution, and the presence of open water or thin ice leads). In addition, 24 hr predictions of regional ice drift velocity and direction provided daily by Drift+Noise Polar Services GmbH/AWI aided in positioning the vessel (SIDFEx, 2022). These ice maps and ice-drift models covered an area of 360 km2 around the Aurora mound and were useful for predicting when open-water leads would be positioned above the vent field (Figure 2b). With a range of six nautical miles, the vessel’s ice radar aided navigation among ice floes.
ROV Operations
ROV Aurora is a Kystdesign SUPPORTER 32-type ROV (Figure 2c) owned and operated by REV Ocean. It has a 6,000 m depth range and is fully equipped with a state-of-the-art science skid. Importantly for under ice operations, Aurora is configured to operate as part of a two-bodied system that comprises a separate tether management system (TMS) called Borealis, with an additional 1,000 m of neutrally buoyant tether. For protection against adverse interactions with sea ice, the ROV was deployed through the moonpool of the Norwegian icebreaker R/V Kronprins Haakon. The Aurora Borealis system descended as a unit toward the seafloor, which permitted a rapid descent (0.8–1 m s–1) of the vehicle while the vessel was drifting with the ice, avoiding tension on the ROV’s neutrally buoyant optical tether (Figure 3). When the vehicle was at 50–100 m above seafloor, depending on topography, the winch was stopped and the ROV deployed from its TMS. Safe ROV operations to the Arctic deep seafloor were only possible during this expedition because of the two-bodied configuration of the ROV and the availability of a moonpool for deployment and recovery operations. The soft umbilical of the TMS enables the ROV, once out of its TMS, to fly faster than the boat drifts with the ice. This allows the ROV to reach the target before the vessel drifts over it, providing additional time for the ROV to work on the seafloor on a small target such as a vent field (Figure 3). Operations through the moonpool protected the ROV and its TMS from ice contact and ensured that an open area was always available for the recovery of the ROV. The operational methodology described below aims at maximizing seafloor time on a small target (in this case, the active area of the Aurora Vent Field, only about 75 m2) at great depth (4,000 m) under drifting ice. If the goal was to conduct a survey in a region without a precise geographic target (e.g., a biological or sedimentological transect in a region, to sample opportunistically), vessel positioning and ice drift velocity and direction would be less critical.
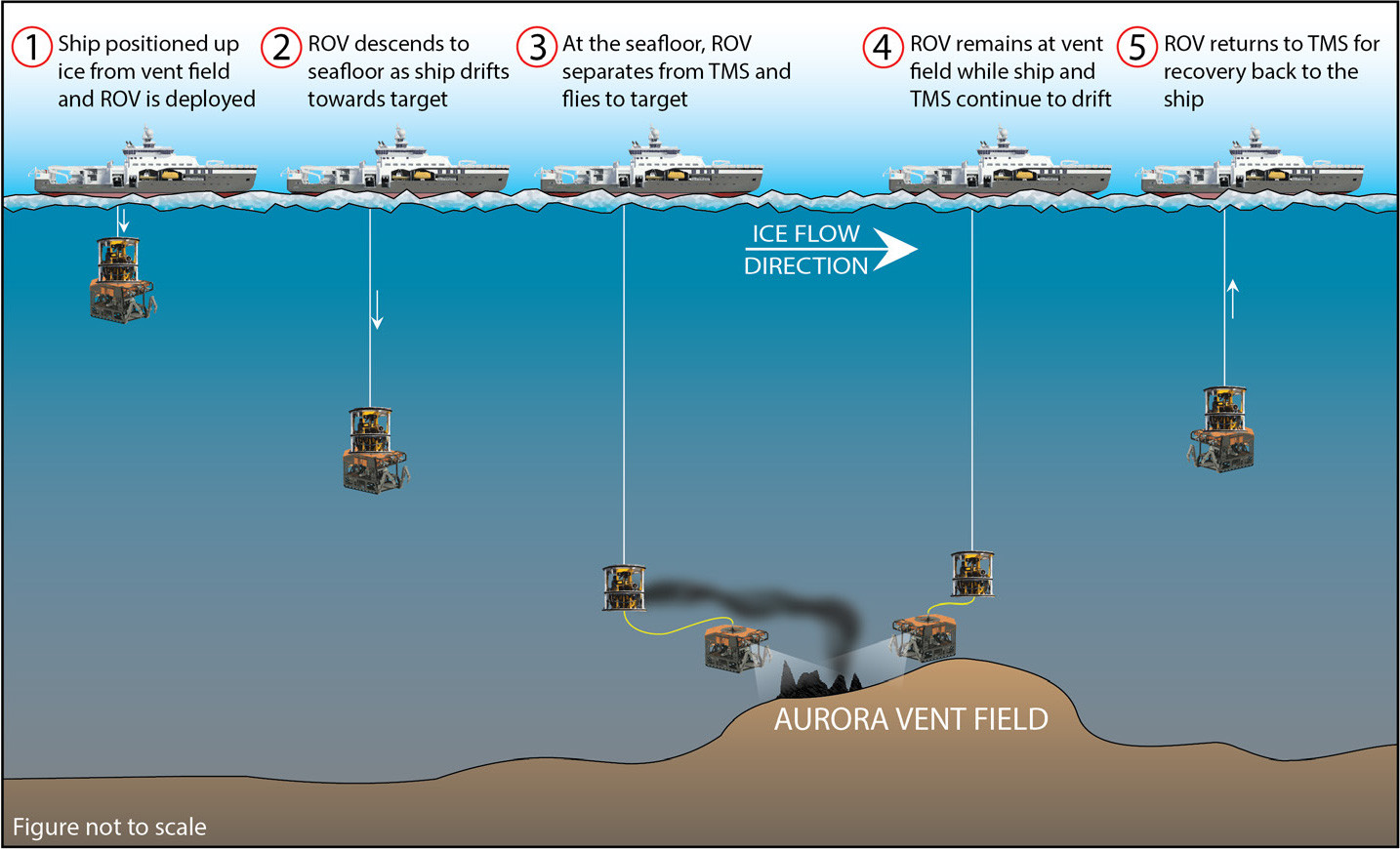
FIGURE 3. Operational sequence for ROV dives on small deep seafloor targets under drifting ice. > High res figure
|
For the purpose of studying the AVF, once a suitable position for the vessel was determined, the speed and accurate direction of the ice floe were assessed by positioning the vessel against the ice floe and drifting with it for 15 minutes. Timing for ROV deployment could then be based on the drift speed. ROV Aurora was launched at a distance from the vent field that allowed the vehicle to reach the seafloor upstream from the study area and fly ahead of the vessel toward the vent field. Drift speeds up to 0.3 knots provided sufficient bottom time (1–2.5 hr) for the ROV to work on the seafloor while stationary at a target location. Operations between 0.3 knots and 0.6 knots were feasible but challenging, and seafloor time at the study area was very limited (20–40 min). Above 0.6 knots, the risk of operations was considered too high and the ROV was not launched. If the distance between launch and study area was too large, the probability of the ice drift changing direction during the ROV’s descent, taking the vessel away from the target area, was higher, particularly with slower ice drift <0.1 knot. If the launch position was too close to the vent field, there was not sufficient time for the ROV to reach the seafloor before the vessel drifted beyond the study site and the ROV had to be recovered. Of the 14 times ROV Aurora was launched over the AVF, the dives had to be aborted on six occasions due to a change in ice drift direction and/or speed that took the vessel off-track from the vent field. On one occasion, the drift direction changed from south to southeast, and then east-northeast within hours, which resulted in the ROV being launched and recovered four times before we were able to complete a successful dive.
The Aurora Vent Field
Geological and Geochemical Settings
The Aurora Vent Field is located on the southwest part of the Aurora mound (Figure 1b,c). It consists of pillow basalts and active and inactive vents, and encompasses an area of ~140 m × 100 m. There are steep-sided inactive hydrothermal edifices up to several meters high, individual actively venting chimneys, and abundant hydrothermal crust and chimney debris that, along with the pillows, are variably covered by as much as ~3 m of sediment.
Current hydrothermal activity is restricted to near the center of the AVF (82°53'49''N, 6°15'21''W) and consists primarily of three isolated, vigorously venting black smoker chimneys that occur in a ~10 m diameter cluster. These three active black smokers have been named Hans Tore, Enceladus, and Ganymede (Figure 4a,c,e). The Hans Tore vent was observed with OFOBS during HACON19 (Bünz et al., 2019). It was named in memory of Professor Hans Tore Rapp (Figure 4b), colleague and friend from the University of Bergen, who passed away in 2020. Prof. Rapp had been involved in the 2014 AURORA PS86 and HACON19 missions as fauna expert and was a key figure in the development of the HACON project. The two other smokers are named after “ocean world” moons in the solar system, some of which may host hydrothermal activity with the potential to harbor associated chemosynthetic-based life. Saturn’s ice-covered moon Enceladus (Figure 4d) was chosen because its ocean is suspected to be many tens of kilometers deep and predicted to host hydrothermal activity (Cable et al., 2021). Ganymede, an ice-covered satellite of Jupiter (Figure 4f), is the largest moon in the solar system and may have the largest saline ocean—an ocean that may be implicated in altering its polar aurora (Saur et al., 2015), providing a fitting connection to this locale.
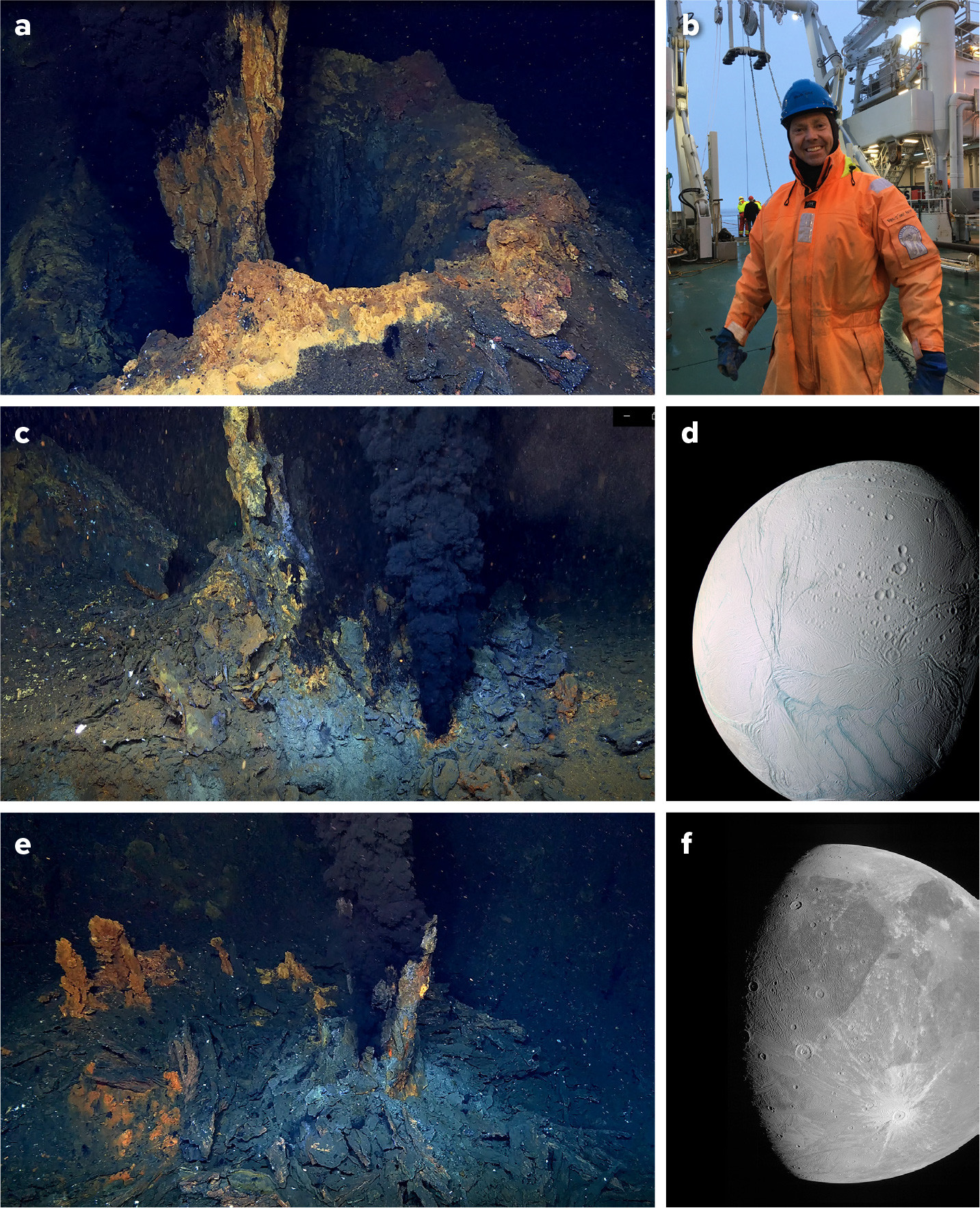
FIGURE 4. Aurora Vent Field black smokers and their names: (a) Hans Tore vent. (b) Professor Hans Tore Rapp during the HACON cruise in 2019. (c) Enceladus black smoker. (d) Enceladus moon. Credit: NASA, Cassini Mission (e) Ganymede black smoker. (f) Ganymede moon. Credit: NASA, Juno Mission. > High res figure
|
The Hans Tore vent (Figure 4a), found at 3,883 m depth, features a tall (at least 2 m), narrow chimney vigorously emitting black smoke fluid from its summit and a second, 1 m tall active chimney next to it. They sit in the middle of a 2 m diameter, 1 m deep circular crater, and black smoker fluid issues from the bottom of the crater through two small chimneys. Diffuse, lower-temperature venting occurs around the rim of the crater, and small oxidized iron-rich chimneys are evident at the rim. At the Enceladus vent (Figure 4c), ~10 m southwest of Hans Tore, a single 1.5 m tall chimney structure emits black vent fluid along its entire length and from its top. It is surrounded by a forest of inactive chimneys at 3,887 m depth.
The Ganymede vent (Figure 4e) is located at 3,884 m depth, ~5 m northeast of Enceladus, slightly south of a line between Enceladus and Hans Tore vents. This black smoker rises on a relatively flat seafloor and consists of black smoker fluid venting from five individual exit orifices at the base or sides of a partially collapsed chimney structure. Both Enceladus and Ganymede are characterized by thin walls, resulting in frequent collapse and regrowth, as evidenced by their relatively short heights and ramparts of abundant collapsed chimney debris.
The chimneys and larger hydrothermal accumulations at the AVF were sampled with the ROV manipulator (Figure 5a). The rocks collected are generally dark greenish-gray and composed primarily of chalcopyrite, pyrite, sphalerite, and barite. The sulfide minerals exhibit varying degrees of oxidation associated with prolonged exposure to seawater (Figure 6a). Samples were also collected from several low-lying orange-brown oxidized iron-rich chimneys associated with lower-temperature diffuse flow, especially those surrounding the rim of the Hans Tore vent crater. The formation of these extremely friable iron-rich deposits has been associated with microbial activity (Johannessen et al., 2020). Post-cruise analyses of the rock samples will be integrated with sediment geochemistry data sets and used to characterize the composition, age, and evolution of the vent field and to assess the relationship between the composition and age of the deposits and the animals and microbes that colonize them.
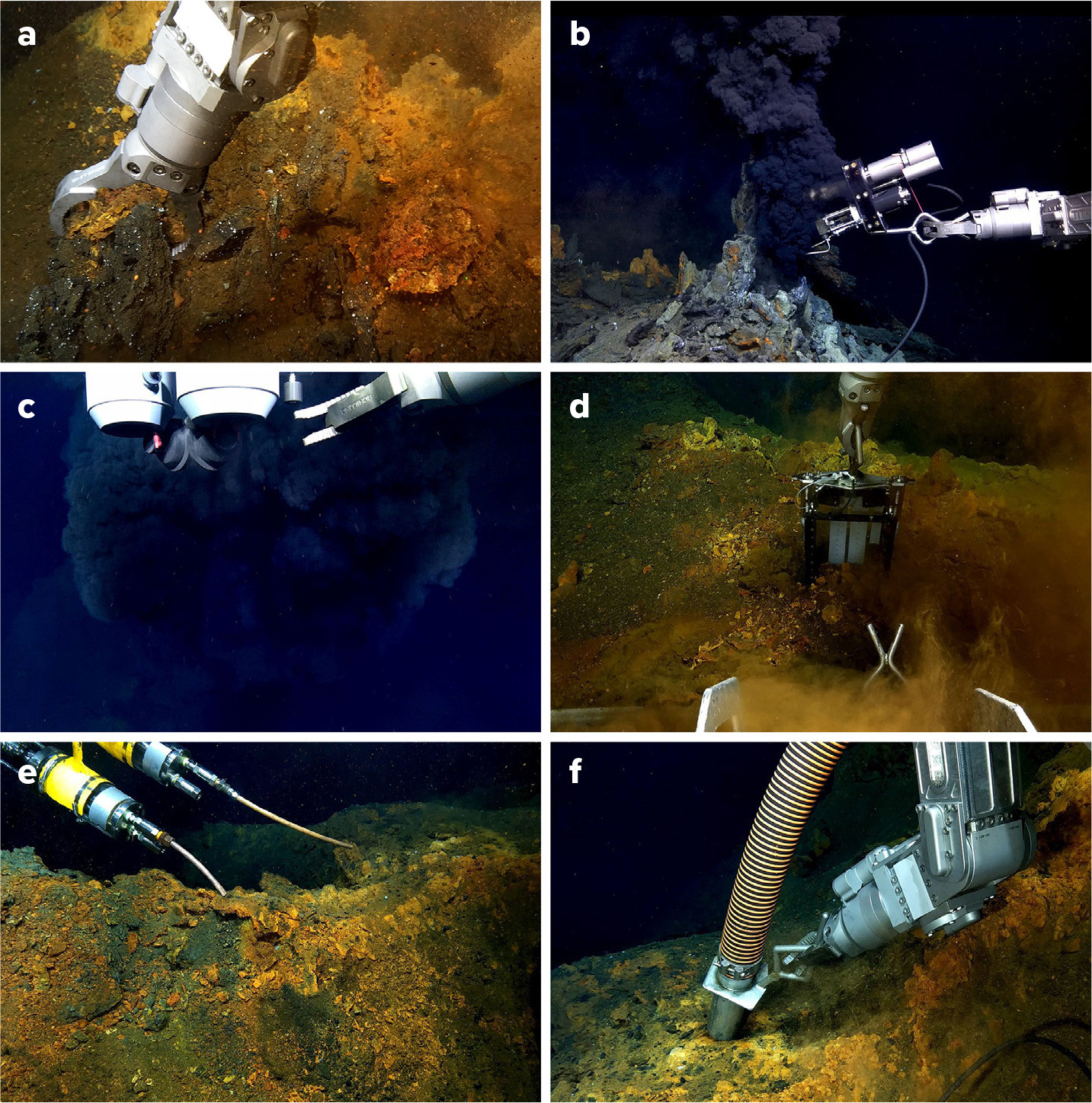
FIGURE 5. Sampling on the Aurora Vent Field. (a) Rock collection with the manipulator on the flanks of the Hans Tore vent. (b) Isobaric gas-tight (IGT) sampling at Ganymede. (c) Plume sampling with Niskin bottles on the Hans Tore vent. (d) Blade core sampling on the side of the Enceladus black smoker. (e) Sampling bacterial mats with the biosyringe on the rim of the Hans Tore vent. (f) Suction sampling for amphipods on the flanks of the Hans Tore vent. > High res figure
|
The Enceladus and Ganymede black smoker “end member” fluids and their stable maximum temperatures were each successfully sampled with duplicate isobaric gas-tight (IGT) samplers (Seewald et al., 2002; Figure 5b) for comprehensive inorganic-organic geochemical and stable isotope characterization (Reeves et al., 2011, 2014); “paired” chimney material was also examined. Sufficient static video footage (several minutes) of at least one of the vents was also taken to allow for particle image velocimetry (Mittelstaedt et al., 2012) that will enable first estimates of fluid mass, chemical, and heat fluxes from the vents. The ongoing work will be critical for deciphering the types of chemical compositions venting at Aurora and how they support the ecosystem. The data will also provide information on the water-rock interactions between hydrothermal fluids and the underlying crustal substrate (e.g., Reeves et al., 2011, 2014), allowing direct comparison of the chemical energy “landscape” of AVF fluids (e.g., Dahle et al., 2015) with other vent sites along the global mid-ocean ridge system.
Hydrothermal fluids discharged at the seafloor form neutrally buoyant mid-water plumes that disperse horizontally along isopycnals, transporting and redistributing metals across entire basins (Resing et al., 2015). Correlating paleo-plume events recorded in the sedimentary record therefore enables us to provide spatial and temporal constraints on vent activity and the contribution of seafloor vent metal exports to deep ocean budgets (Antonelli et al., 2017). Hydrothermal plumes also transport trace elements, nutrients, dissolved gases, and vent-derived biomass that can support heterotrophic deep-sea communities (Cathalot et al., 2021; Levin et al., 2016). At the AVF, the dispersing plume was identified from water-mass turbidity measured during CTD casts. The buoyant plume was located in the bottom layer between 3,000 m and 4,000 m depth during the AURORA (Boetius, 2015; German et al., 2022), HACON19, and HACON21 cruises. Dissolved methane concentrations in water samples collected from ROV-mounted Niskin bottles during HACON21 (Figure 5c) at ~10 m directly above one of the vents were measured using headspace gas chromatography (e.g., Sert et al., 2020). In these samples, elevated dissolved methane in the near vent buoyant plume (~102–103 nM) decreases dramatically to 30 nmol/L in the immediately overlying non-buoyant plume (German et al., 2022a), 1–10 nmol/L in the more distal plume (Sert et al., 2022), and eventually to background concentrations (~<0.5 nM). This shows that strong methane gradients that prevail in the vicinity of the rising buoyant plume could support chemosynthetic microbial activity in the water column (Anantharaman et al., 2013). In buoyant and non-buoyant plumes, methane dilution, oxidation, and methanogenesis may occur simultaneously (Ver Eecke et al., 2012), contributing to deep-sea biogeochemical cycling.
Biological Communities of the Aurora Vent Field
Biological communities in hydrothermal systems are typically driven by chemosynthetic microorganisms acquiring energy from the oxidation of reduced compounds in hydrothermal fluids (e.g., sulfide, methane, hydrogen) and oxidized compounds in seawater (e.g., sulfate, nitrate, oxygen) (Van Dover, 2000). The availability of different chemical energy sources between and within vent systems form chemical energy landscapes that shape microbial communities and possibly those of microbial primary production grazers (Dahle et al, 2015). Deciphering geo-biological connections in hydrothermal systems requires detailed analyses of the composition of fluids along with analyses of microbial community structure and functioning. Samples for microbiological analyses were obtained from active and inactive chimneys, low-temperature diffuse flow sites, and background sediments. The rocks collected for geological studies (Figure 5a) were firstly sub-sampled on board for their microbiota. The blade core was used to sample undisturbed sediment samples (Figure 5d), and biosyringes were used to sample microbial mats (Figure 5e). Ongoing geochemical and metagenomic-based community analyses will shed further light on the AVF microbial community structure and functioning and the biogeochemical relationships between sediment substrate (grain size, mineralogy, and geochemical composition) and microbial and faunal communities. The results will reveal to what extent the unique setting of the AVF stimulates development of unique microbial assemblages and ecosystems.
Infauna from sediments recovered from the vent field with the blade core (Figure 5d) and from a reference site a few kilometers off vent with a ship-operated multicorer were sectioned at different intervals, sieved with 1,000-, 500-, 250-, 150-, and 32-micron mesh sieves and identified under a microscope in the laboratory. The infauna was composed, predominantly, of meiofaunal taxa (32 µm to 1,000 µm), specifically free-living nematodes and foraminifera. These groups are known to be a key link in the deep-sea food web, including in hydrothermal vent and cold seep environments (Zeppilli et al., 2018). Classical abyssal polar meiofauna communities in sediments a few kilometers away from the AVF are represented by opportunistic species dependent on bacterial and phytodetrital matter, including low-energy species (Vanreusel et al., 2000). Meiofaunal community densities and diversity in hydrothermal-derived sediments were depleted when compared to communities within reference sediments off vent. Benthic foraminifera in these hydrothermal-derived sediments, however, include organic-walled allogromids that seem to tolerate reduced and low amounts of organic carbon microhabitats. These initial results suggest a critical role of the meiofauna in the vicinity of vents for biodiversity and potential bio-indicators for ecosystem health in vent fields.
Samples of macro- and megafauna were obtained from chimney debris collected with the ROV manipulators (Figure 5a), from the sediment surface collected with the suction sampler (Figure 5f), and from sponge aggregations at the vent periphery (Figure 6f). Preliminary analyses show an increased presence of molluscs, crustaceans, and polychaetes in the AVF compared to the surrounding rock fields. Within the molluscs, a new species of cocculinid limpet, Cocculina aurora sp. nov. (Chen et al., 2022; Figure 6b) and two species of gastropods of the families Rissoidae and Skeneidae (Figure 6c) were the most abundant taxa found on the rocks. Samples collected with the suction sampler targeted amphipods, tentatively identified as belonging to the family Melitidae (Figure 6d,e), the second most dominant group at the active vent field after the molluscs.
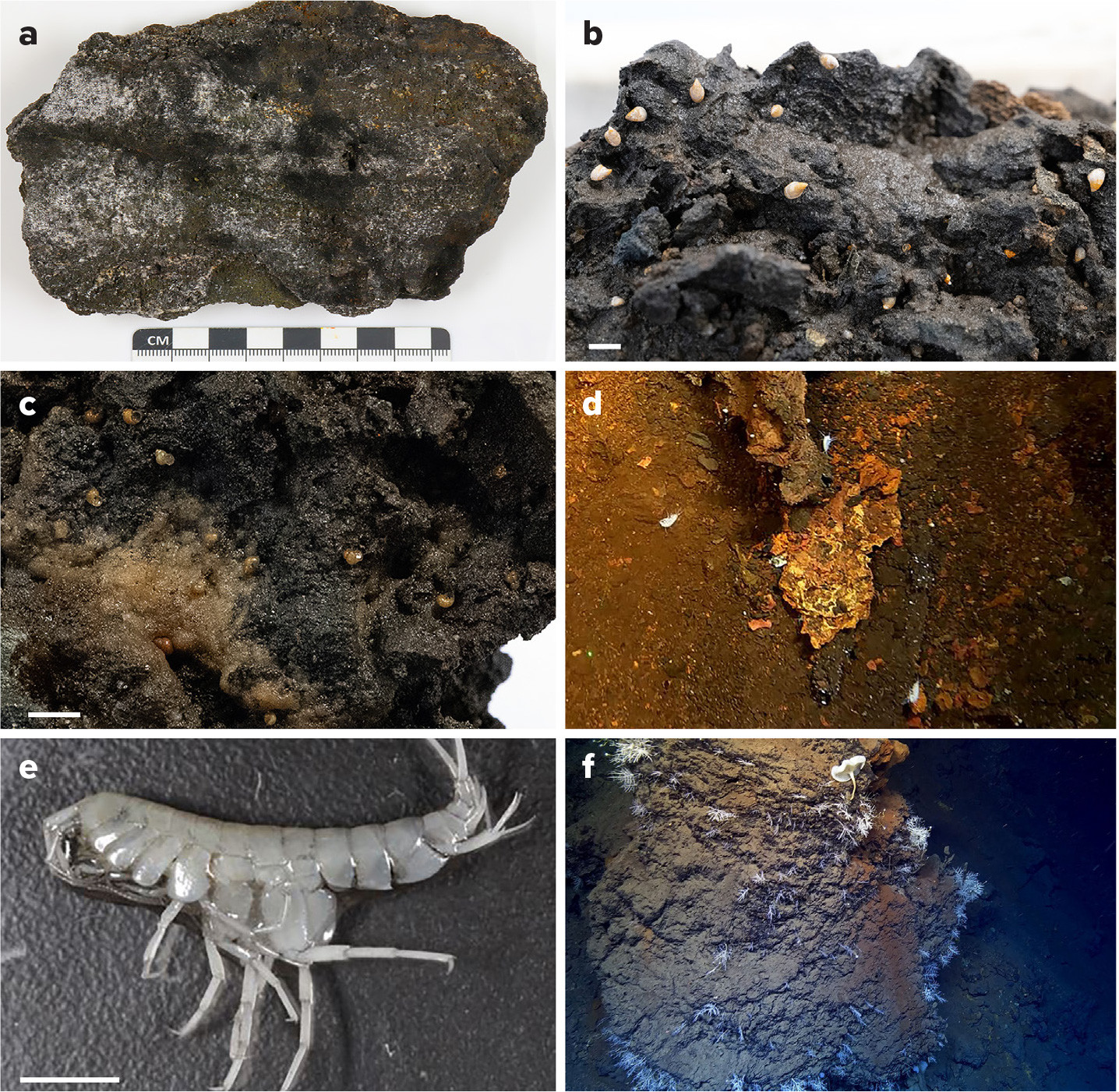
FIGURE 6. Samples from the Aurora Vent Field. (a) Representative chimney sample collected from the Enceladus black smoker, composed primarily of a mixture of sulfide minerals (chalcopyrite, pyrite, sphalerite) and barite. (b) Rock hosting Cocculinid limpets and gastropods (scale bar = 5 mm). (c) Detail of the rock in B showing the high abundance of gastropods (scale bar = 5 mm). (d) Amphipods on the rim of the Hans Tore vent. (e) Close-up of an amphipod in the lab (scale bar = 5 mm). (f) Cladorhizid carnivorous sponges on the periphery of the Aurora Vent Field. > High res figure
|
At the Hans Tore vent, amphipods and small gastropods can be seen on the rim and outer slopes of the crater. At Enceladus and Ganymede, amphipods are evident on the chimney walls, whereas gastropods are mostly present on chimney debris at the base of the black smokers. Suction sampling at the Hans Tore and Ganymede vents also retrieved a few specimens of polychaetes and some empty polychaete tubes. The AVF vent fauna, dominated by small gastropods and amphipods, is similar, on a higher taxonomic level, to the faunal communities of the Loki’s Castle Vent Field at 73.5°N on the Mohns Ridge (Pedersen et al., 2010). Further work on the taxonomy, connectivity, and biogeography of the fauna of the AVF is ongoing in order to clarify the links between the AVF fauna and that of other vent fields. In contrast to the Mohns Ridge vents, sedimented areas with diffuse venting were not observed, which explains the restricted spatial distribution of vent-adapted fauna close to the black smokers. Basalt outcrops within the vent field host dense aggregations of carnivorous sponges belonging to the family Cladorhizidae (Figure 6f). Even though these organisms are typical representatives of the background fauna, they can thrive at higher densities in the vicinity of active vents by possibly taking advantage of the food-enriched environment driven by the hydrothermal activity, while tolerating increased abiotic stress (Levin et al., 2016; Georgieva et al., 2020).
A comprehensive ROV video survey was also conducted to provide visual data for mapping the different habitats across the AVF and to help decipher differences in communities detected by the OFOS and OFOBS photo transects across the Aurora mound and further sites along the Gakkel Ridge (Boetius, 2015; Bünz et al., 2019). The video analyses will provide information on the distribution, abundance, and density of the different species across the three AVF black smokers and their geochemical gradients (Schoening et al., 2012). As previously reported (Boetius, 2015; Bünz et al., 2019), rocks, pillow lavas, and basaltic ridges and outcrops in the proximity of the vent field were covered by dense aggregations of the hexactinellid sponges Caulophacus arcticus and Asconema megaatrialia. These species are common inhabitants of deeper areas in the Nordic Seas (Roberts et al., 2018), and the unusually high densities found at the Aurora mound compared to non-venting mounds on the Gakkel Ridge (Boetius, 2015) suggest that the higher productivity around the active vent field provides increased food supply that can support higher sponge density. Aggregations of sponges and dead stalks of C. arcticus may have a crucial facilitating role for other fauna, since bythocarid shrimps, anemones, crinoids, and several species of isopods and amphipods seem to be more abundant in areas covered by hexactinellid sponge habitats.
Although a detailed biogeographic analysis that includes the fauna from the Aurora Vent Field is underway, the currently available observations provide insights into the position of the Gakkel Ridge vent communities in global vent biogeography. Six biogeographic regions of vent fauna defined by Bachraty et al. (2009) were increased to 11 regions of biogeography when the data were reanalyzed, incorporating the vent fields of the Southern Ocean (Rogers et al., 2012). However, none of these studies included data from Arctic latitudes. Our observations of the Aurora Vent Field suggest faunal similarities with the Loki’s Castle Vent Field at 73°N (Pedersen et al., 2010) in terms of the presence of amphipods and gastropods as main groups, but also show differences such as the lack of siboglinid tubeworms and the presence of the new species of limpet Cocculina aurora sp. nov. (Chen et al., 2022). The evolution and genetic connectivity of fauna from the deep Central Arctic Ocean is a major unknown, and the data from the HACON project will contribute to addressing it. The limited knowledge of Central Arctic Ocean deep-sea ecosystems is a direct consequence of the operational challenges of working at great depths under drifting ice, which heavily constrains our capacity to develop robust management measures and monitoring procedures for a pristine region prior to the expected increase in industrial activity as the Central Arctic Ocean opens to human activities.
The Ice-Covered Arctic as a Bridge to the Search for Life in Space?
A particularly exciting aspect of the discovery of hydrothermal vents beneath an ice-covered ocean is its impact on humanity’s search for life beyond Earth. The collection and analysis of 18 full sea-ice cores ranging from 98 cm to 181 cm in length, as well as three gray ice cores ranging from 8 cm to 15 cm, served the dual purpose of advancing our understanding of sea-ice processes on Earth and providing a useful analog for investigating the surface chemistry of distant ice-covered ocean worlds elsewhere in our solar system. On Earth, the seasonally variable sea-ice cover modulates the exchange of gases between the ocean and the atmosphere. During the winter period of maximum coverage, and of sea-ice growth, gases such as carbon dioxide and methane cannot escape directly to the atmosphere, but rather are captured in the ice matrix. Similarly, gases from the atmosphere cannot reach the ocean, as mixing is inhibited by the ice layer. In addition, some buoyant materials, such as organic lipids and other biological materials, can become entrained in the ice matrix. The seasonal exchange efficiency of gases in the Arctic, especially carbon dioxide, is a poorly constrained variable in our efforts to model and understand climate change. We conducted analyses of trapped carbon dioxide and methane in many of the cores while onboard R/V Kronprins Haakon and have generated depth profiles of gas concentration that show the detailed transition from atmospheric to oceanic concentrations of carbon dioxide and methane. In addition, we measured the 13C concentration in methane, which we can use to differentiate between biogenic and abiogenic contributions to the methane signature.
Connection of this work to distant ice-covered moons, such as Jupiter’s Europa, is based on the knowledge that Europa’s ice shell is essentially ~10 km of sea ice (Figure 7). Ocean salts, carbon dioxide, and other materials have been observed spectroscopically on the surface of Europa (Hand et al., 2007; Hand and Carlson, 2015; Trumbo et al., 2019). The best analogous systems on Earth to Europa’s ice are the sea ice of the Arctic and the Antarctic. While the scale of ice thickness is much different, the processes by which gases and organic materials, including microbes, are incorporated into sea ice on Earth can inform the development of missions and instruments designed to search for such materials either remotely or with a landed spacecraft on Europa. To this end, our team processed seven ice cores for microbiology primarily to determine whether any thermophiles that may have been transported via hydrothermal plumes are captured in the ice cores. This is an interesting microbial ecology question in its own right, but it also carries interesting implications for the search for biosignatures on Europa, as the seafloor there could be hydrothermally active, and plumes could deliver material to the ice-water interface.
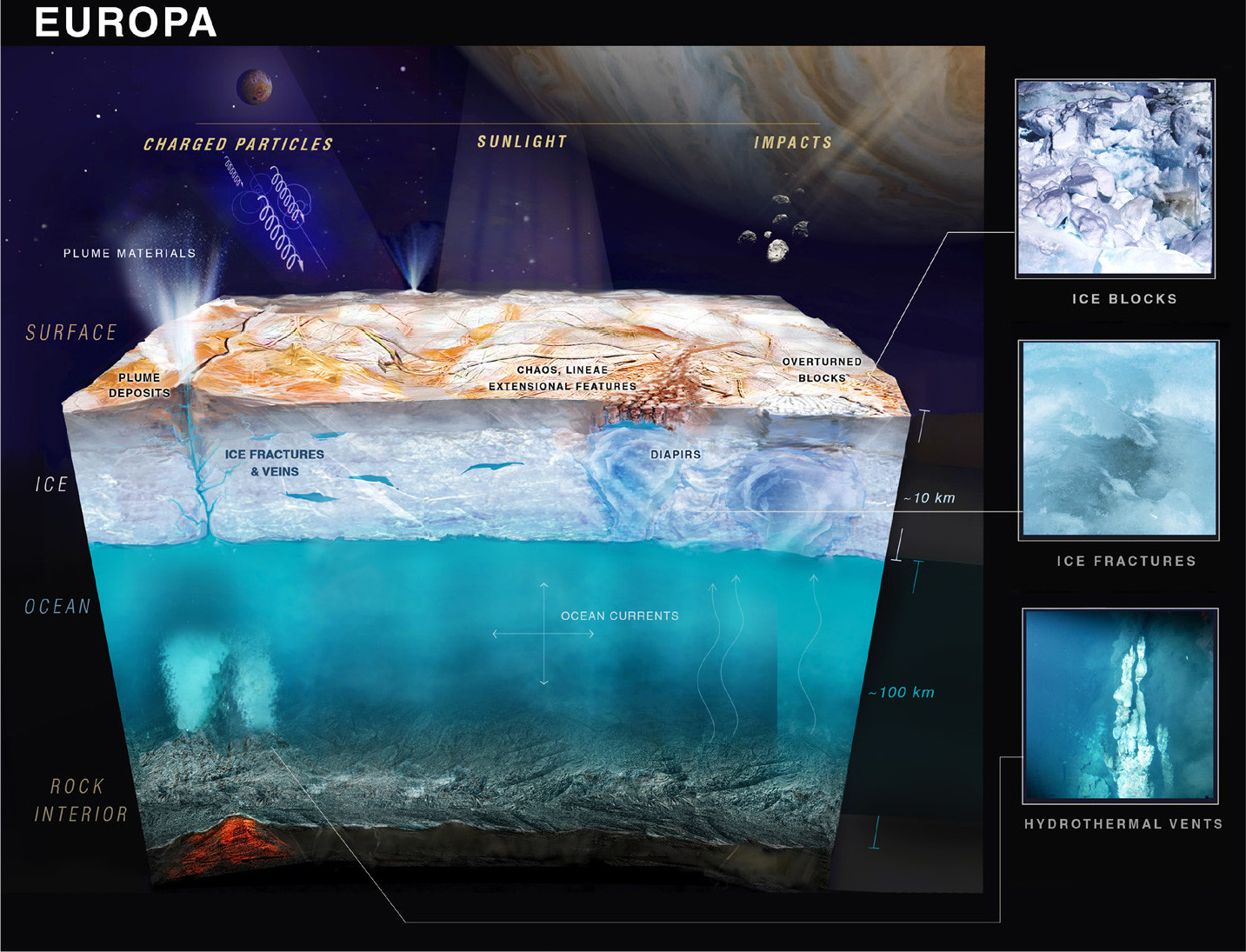
FIGURE 7. While the physical scale and thickness of the ice differs greatly between Earth’s sea ice and that of Europa’s ice shell, both icy environments serve as a window into the oceans hidden below. On Earth, we can study the ice, ocean, and seafloor to reveal any processes that provide connections across these interfaces. On Europa, we are, at least for the near future, limited to using the surface chemistry of the ice as our primary means for understanding the ocean and seafloor chemistry and geology. Image courtesy NASA/JPL and the 2017 Europa Lander Science Definition Team Report (Hand et al., 2017). > High res figure
|
Since the first demonstration that a saltwater ocean must be present beneath the icy exterior of Europa, we now know of multiple salty-ocean worlds in our own solar system, including Jupiter’s other moons, Ganymede and Callisto, and Saturn’s moons Titan and Enceladus (Hand et al., 2020). Among them, those that have rocky seafloors in contact with salty oceans are of particular relevance. The discovery of chemosynthesis at vent sites beneath the ice-covered Arctic Ocean now allows us to articulate the case for why icy moon oceans could be inhabited, even if they are too far removed from sunlight to sustain photosynthesis (Hand and German, 2018; German et al., 2022b). Of course, animal life as we know it depends on oxygen, which is the result of photosynthesis on Earth, but some of the microbes detected in the Aurora vent plume relate to microaerophilic or even anaerobic types (Massimiliano Molari, Max Planck Institute for Marine Microbiology, pers. comm., 2022). The intellectual challenge in pursuing this line of research becomes more compelling when considering that a significant proportion of the exoplanets that have now been discovered orbiting other stars may also be ocean worlds (Quick et al., 2020). A next critical step in that exploration will begin soon, with the 2023 launch of the European Space Agency’s JUpiter ICy moons Explorer (JUICE) expedition to investigate Europa and Ganymede and the 2024 launch of NASA’s Europa Clipper mission to study that body’s habitability. In the longer term, planning has already begun for future landed missions to search for evidence of life on both Europa (Hand et al., 2022) and Enceladus (MacKenzie et al., 2021).
Acknowledgments
We would like to thank the captains, officers, and crew of R/V Kronprins Haakon and R/V Polarstern, the teams of ROV Aurora, ROV/AUV NUI, and OFOBS, as well as all participants in the cruises for their invaluable contributions while at sea. The HACON project was funded by the Norwegian Research Council (grant # 274330). The AURORA mission (PS86 2014) was funded by the Helmholtz Association, the MARUM DFG Cluster of Excellence at the University of Bremen (49926684), and the ERC Advanced Investigator Grant ABYSS (294757) to AB. CA, PAD, BF, GP, MFS, MS, KAW, and SB were supported by the Research Council of Norway (RCN) through its Centres of Excellence funding scheme (project no. 223259). CRG acknowledges additional support in the USA from NOAA’s Office of Ocean Exploration and Research (Grant #’s NA14OAR4320158, NA19OAR0110406). CRG and KPH acknowledge NASA’s Astrobiology Program (Grant #’s NNX16AL04G, NSSC19K1427). Identification of macrofauna was supported by the project “Fauna of hydrothermal vents and cold seeps in Norwegian waters” funded by the Norwegian Biodiversity Information Centre (the Norwegian Taxonomy Initiative). AH and SPR work was supported by funds from FCT/MCTES granted to CESAM (UIDP/50017/2020+UIDB/50017/2020+LA/P/0094/2020). SPR acknowledges additional support from the FCT/MCTES, through the “CEEC Individual 2017” contract (CEECIND/00758/2017). LV was supported by the Norwegian Research Council project number 287934. PAR work and genetic connectivity studies were supported by the project “Eco-Safe” funded by the Research Council of Norway (project no. 326881). EPR work on chemical, isotopic and organic characterization of AVF fluids was additionally supported by the project “HyPOD” funded by the Research Council of Norway (project no. 287364).