Motivation
The Roger Revelle Commemorative Lecture provides a unique opportunity to share an ongoing, decade-long journey toward the goal of biology-inspired ocean exploration, a concept we introduce in this article. The ocean is, in many regards, Earth’s final frontier, and its exploration presents an attraction that no doubt also called to Roger Revelle himself, as he dedicated much of his career to furthering oceanographic research and studying climate change.
From working at the Scripps Institution of Oceanography to serving on the Scientific Committee on Oceanic Research (SCOR)—among a multitude of other leadership roles in academia and policy—Revelle demonstrated a fundamental commitment to expanding our knowledge of the ocean. His dedication to both basic and applied research led to advancements on international scales, and in particular, to the introduction of the concept of climate change through studies of the chemical makeup of the ocean. The “Revelle factor,” defined as the ratio of instantaneous carbon dioxide (CO2) and total dissolved inorganic carbon (Broecker et al., 1979), is a metric of the ocean’s absorption of atmospheric CO2 that is still actively used, among other buffer factors, in biogeochemical research for tracking the chemistry of the ocean (Egleston et al., 2010; Cai et al., 2020; Humphreys et al., 2022). In fact, Revelle’s work with Hans Suess regarding atmospheric CO2 and dissolved inorganic carbon led to the first reported use of the term “global warming” (Revelle and Suess, 1957). Now known by some as the “father of global warming,” Revelle conducted studies measuring climate change, spoke about potential anthropogenic causes, and chaired formal study groups, such as the Committee on Climate Change and the Ocean (CCCO) within SCOR and the Intergovernmental Oceanographic Commission of the United Nations Educational, Scientific, and Cultural Organization (IOC-UNESCO; Sabine et al., 2010).
To fully appreciate the significance of these studies of the ocean and associated climate change impacts, we must remember that the ocean represents approximately 99% of the habitable volume on Earth (NASA Science: Share the Science, 2022), and it is estimated to serve as home to over two million different species of organisms (Mora et al., 2011). In addition to its complex ecosystems that host a wealth of biodiversity, the ocean is recognized as a primary lever of climate change, for example, via its ability to sequester carbon (DeVries et al., 2019). And, in terms of the world economy, up to 6 trillion USD per year can be attributed to the ocean—through shipping, fisheries, energy, tourism, and a variety of other economic activities (Gillsater, 2018)—with over 90% of global trade reliant on sea routes (OECD, 2020). Furthermore, ocean-based industries disproportionately impact developing countries, with six marine industries contributing to over 11% of the gross domestic product (GDP) in lower middle-income countries and 6% in low-income countries, compared to less than 2% in high-income countries and members of the Organization for Economic Co-operation and Development (OECD). In some low-income countries and small island developing states, ocean-based industries accounted for over 20% (OECD, 2020). On these bases alone, it is not an overstatement that the future of life on Earth depends on sustainable ocean stewardship.
And yet, by multiple measures, the ocean remains relatively poorly studied and relatively poorly understood. What we do know is quite literally a drop in the ocean. Quantifying this knowledge gap is challenging a priori, but it can be illustrated by comparing our knowledge of ocean bathymetry (i.e., topological studies of the ocean floor and seabed structures) to our knowledge of the structure and dynamics of other planets. Today, approximately 10%–15% of the seafloor has been mapped at 100 m resolution, as compared to 100% of our neighboring planets Mars and Venus (Copley, 2014). To support the effort of ocean mapping here on Earth, in 2017 The Nippon Foundation and the General Bathymetric Chart of the Oceans (GEBCO) Seabed 2030 Project began an effort to map the entire ocean floor, as part of the United Nations (UN) Decade of Ocean Science for Sustainable Development (2021–2030; https://www.oceandecade.org/). Compared to the GEBCO grid map in 2021, an additional 2.8% of the ocean floor has been mapped, bringing the estimate of the total seafloor mapped to 23.4% in 2022, ranging from 100 m to 800 m grid sizes (https://seabed2030.org/mapping-progress). However, the disparity with our knowledge of neighboring planets becomes even more dramatic if we consider smaller length scales, such as 1 m resolution. These are scales at which many important biological phenomena occur in the ocean, and yet, less than one-tenth of one percent of the seafloor has been mapped at that resolution (Mayer et al., 2018), although there are ongoing efforts through bathymetric surveys (Fregoso and Jaffe, 2020) and crowd-sourcing (Novaczek et al., 2019).
Aside from seafloor mapping, the majority of mid-water regions—approximately one billion cubic kilometers of the ocean—also remain unsampled, with estimates that up to 90% of species biodiversity in the ocean still remains unstudied (Reaka-Kudla, 2001). To date, approximately 240,000 marine species have been catalogued by experts as “accepted marine species” in the World Register of Marine Species (WoRMS Editorial Board, 2022). Moreover, because ocean water masses are not static, sampling a given region one time is likely insufficient to claim a comprehensive understanding of that region of our temporally evolving ocean. With a multitude of such confounding factors, it is difficult to determine how ocean environments develop naturally, as well as to quantify potential anthropogenic forces and their effects. In sum, the UN Ocean Decade faces many challenges (NASEM, 2022).
Existing Tools for Ocean Exploration
To be sure, a suite of increasingly powerful measurement tools has advanced ocean exploration and ocean science over the past several decades. Figure 1a provides examples, from aerial to surface to subsea tools; additional systems are in development and coming online each year. In examining the figure, note the bias of our measurement tools toward the ocean surface. To begin, remote sensing of the ocean uses sensor technologies on satellites and aerial vehicles. These sensors measure physical characteristics, such as spectral radiance, to assess water turbidity, concentrations of phytoplankton, sea surface temperatures and topography, and wave interactions with sea ice (Chapron et al., 2008; Kavanaugh et al., 2021; Collard et al., 2022). Resulting satellite images can be used to map ocean characteristics like phytoplankton blooms. Although remote-sensing data from technologies such as radar or radiometers can offer valuable synoptic measurements across large spatial areas and over relatively long time periods, optical properties of seawater constituents (e.g., absorption and scattering across different wavelengths) limit the water depth to which satellites can provide useful information.
In contrast, in situ ocean measurements take advantage of tools and sensors that interact directly with surface and subsea environments. Surface vessels have served as a primary tool for ocean science for well over a century. Now, to address efforts toward mapping the entire seafloor, autonomous surface vehicles (ASVs) and other surface and subsea measurements are collecting bathymetric data with increased spatial and temporal resolutions (Figure 1b). Furthermore, by deploying instruments such as conductivity-temperature-depth (CTD) probes, it is possible to record the ocean’s salinity and temperature at full depth in many regions of the world. However, it has been estimated that it would require 200 ship-years to sample the entire ocean at just one depth, that is, a single ship moving through the ocean for 200 years, or a fleet of 200 ships, all working in concert for a whole year—just to measure one depth in the ocean (NRC, 1959). In addition to the prohibitive expense of such an endeavor, the dynamic nature of the ocean means that an initial series of measurements might be rendered obsolete by the time a campaign to measure the entirety of a single depth of the ocean had concluded. Therefore, although surface stations, ships, and buoys provide in-depth information for single locations, these tools are limited in their potential to scale to global ocean coverage.
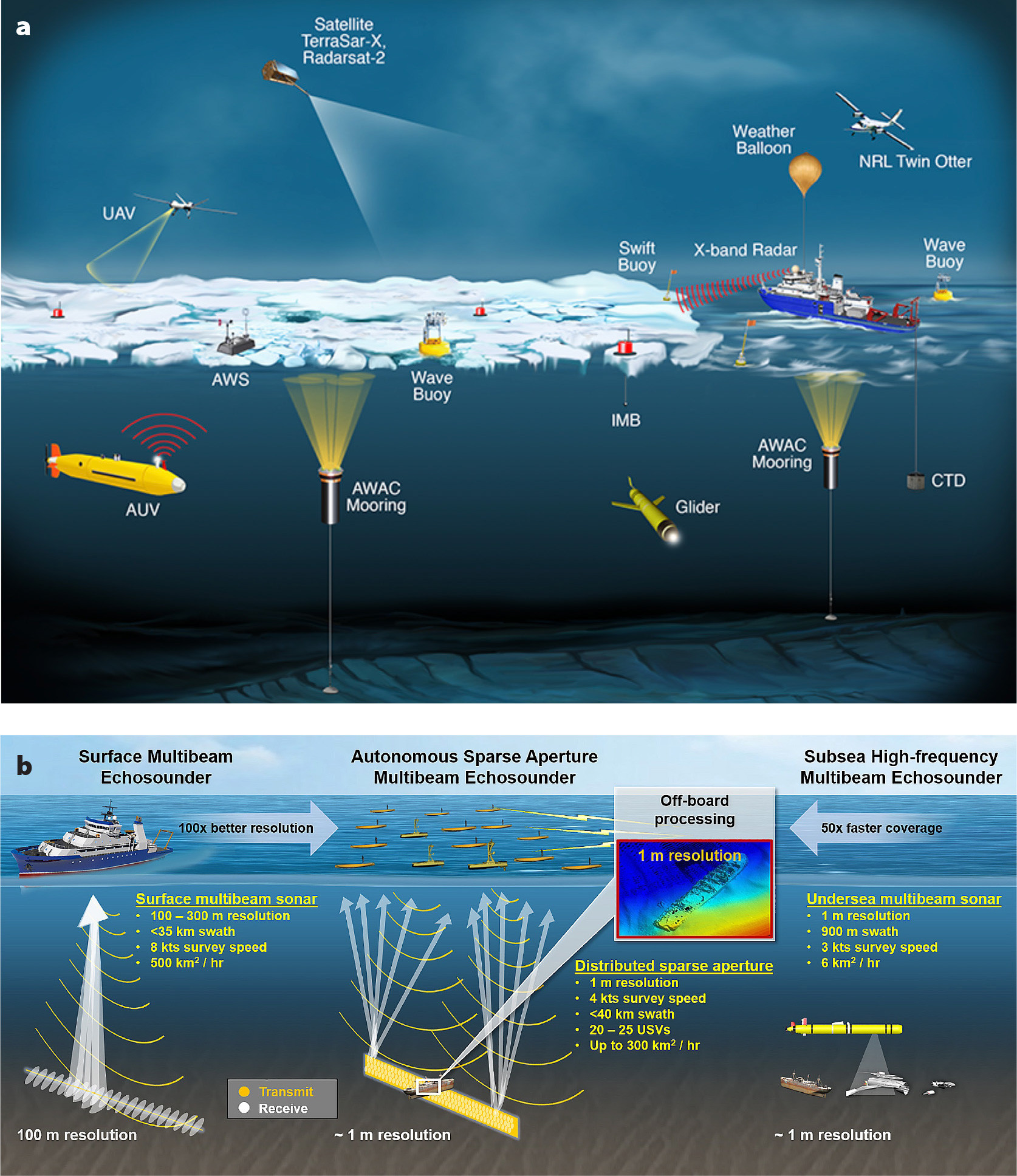
FIGURE 1. (a) Examples of existing tools for studying the ocean, which include imaging radar satellites (i.e., TerraSar-X, Radarsat-2), unmanned aerial vehicles (UAVs), utility aircraft (UV-18 Twin Otter), weather balloons released from ships, Surface Wave Instrument Float with Tracking (SWIFT) buoys, wave buoys, ice mass balance (IMB) buoys, automatic weather stations (AWS), autonomous underwater vehicles (AUVs), acoustic wave and current stations (AWAC), gliders, and ship-based instrumentation such as conductivity-temperature-depth (CTD) instruments. From Thomson et al. (2017) (b) Comparison of three approaches for measuring bathymetry at higher resolutions with faster coverage using multibeam sonar. Adapted from the Lincoln Laboratory at the Massachusetts Institute of Technology. > High res figure
|
Nevertheless, significant progress has been made with efforts using drifters, notably the Biogeochemical-Argo program (BGC-Argo; Biogeochemical-Argo Planning Group, 2016), an extension of the parent Argo program (Roemmich et al., 2009). In addition to profiling the temperature, salinity, and pressure down to 2,000 m depth as do Argo floats, BGC-Argo collects profiles of oxygen, nitrate, pH, chlorophyll a, suspended particles, and downwelling irradiance (Claustre et al., 2020).
A complementary approach to drifters and ship-based measurements is the use of distributed autonomous underwater vehicles (AUVs), untethered systems with onboard power, propulsion, and sensors that operate without real-time input from human operators. These systems are not limited to traversing the ocean surface, but they are typically limited in mission duration due to energy requirements for propulsion, usually from propeller and thruster units (Steele et al., 2009). Current methods of charging AUVs include battery swapping, solar charging, and wired and wireless underwater charging techniques. These generally require that AUVs surface or that personnel manually switch batteries, with the potential risk of exposing electronics to seawater, although recent work is addressing this challenge by using inductive wireless power transfer (IWPT; Teeneti et al., 2021).
Buoyancy- and wave-driven gliders provide another option for ocean exploration with enhanced endurance. These systems can be much more energy efficient than propulsor-based AUVs through use of ballasted pump systems to drive horizontal motion, thus allowing mission durations beyond a full year. However, the more limited maneuvering ability of gliders can inhibit their use near complex seafloor topography (Page et al., 2017). In addition, when the objective is to track naturally occurring biological phenomena in the ocean, the inability of gliders to maintain station without triggering animal avoidance behaviors can create an impediment for studies of biological oceanography.
Additionally, while the costs of existing ocean technologies continue to decline as the associated technologies become more widely available in other commercial sectors, they remain a significant financial investment. For example, each BGC-Argo float costs 100,000 USD for an operational lifetime of four years, and current estimates suggest that an array of 1,000 floats is needed to obtain sufficient data resolution to better understand global biogeochemical processes (Biogeochemical-Argo Planning Group, 2016). Typical cost estimates for propeller-driven AUVs start upward from 50,000 USD, with vehicle maintenance and operational costs resulting in thousands of additional dollars per operating day (Gish, 2004). A single large AUV can cost up to 6 million USD (Claydon, 2006), which is prohibitively expensive to scale to millions of systems. To this end, both commercial and government agencies have dedicated research focuses to building more advanced, cost-effective, and persistent AUVs within the next five to 10 years, ranging from small, portable models to extra-large vehicles such as the Orca Extra Large Unmanned Undersea Vehicle (XLUUV) for various ocean applications (O’Rourke, 2022).
To be sure, the premise that millions of oceangoing underwater systems are truly necessary for comprehensive (i.e., concurrent and global) ocean exploration requires justification. Recall that the volume of ocean water is roughly one billion cubic kilometers. A uniformly distributed array of one million measurement systems would therefore each still be tasked with monitoring an area equal to the size of the city of Los Angeles in the United States (1,000 km2) and throughout a depth of one kilometer of the ocean. By this thought exercise, it becomes apparent that even one million sensors would likely be a significant underestimate for the task at hand. It also underscores the vastness of our ocean. Thus, in addition to further developing the tools described above, new technologies are needed to expand our ocean measurement capabilities and provide information at spatial and temporal scales relevant to ocean science.
A Solution Inspired by Biological Locomotion
One method of creating new ocean technologies is to look to nature for guidance. By using biological principles, we can take advantage of designs that have already been honed through millions of years of evolutionary pressure and, with fewer biological constraints such as evolutionary fitness, potentially improve upon natural designs. Thus, over the past decade, we have explored solutions for ocean exploration that are inspired by the swimming capabilities of many ocean organisms.
Previous research has demonstrated the power of bio-inspired engineering to achieve significant advances in fields as diverse as submarine vehicle design using principles of biological jet propulsion (Ruiz et al., 2011; Whittlesey and Dabiri, 2013), wind energy based on fish schooling behaviors (Whittlesey et al., 2010; Dabiri, 2011), and biomedical diagnostics using flow structure analogues with cardiovascular medicine (Dabiri and Gharib, 2005; Gharib et al., 2006). In the present case regarding underwater vehicles for ocean applications, an important hint regarding the potential for biological inspiration lies in consideration of a performance metric called the cost of transport (COT). This is the energy expended by an organism per unit mass and per distance traveled (i.e., joules per kilogram per meter), which can be considered as the inverse of more traditional efficiency metrics for vehicles, such as propulsive efficiency or fuel economy (i.e., miles per gallon or MPG). Thus, while a more energy efficient car offers higher MPG, a more energy efficient animal exhibits lower COT. Another difference between the COT and more traditional efficiency metrics is the consideration of metabolic costs in COT, which can contribute to lower COT values in some animal species. Figure 2a illustrates COT versus mass for a variety of animals, as well as a representative small AUV (REMUS 100) for comparison. Note that the axes in Figure 2a are scaled logarithmically, which visually diminishes the disparity in the numbers. To underscore this point, Figure 2b visualizes the difference between logarithmic and linear scales. To be sure, the comparison between animals and engineered vehicles is imperfect, as the mass associated with locomotion in organisms is not necessarily that associated with hydrostatic balance in vehicles. Indeed, the dynamics of locomotion at constant velocity are independent of mass (see Newton’s second law). Nonetheless, for the purpose of order-of-magnitude comparison, Figure 2 illustrates the point that the energy efficiency of biological locomotion is competitive with—and may significantly exceed—the efficiency of existing engineering systems.
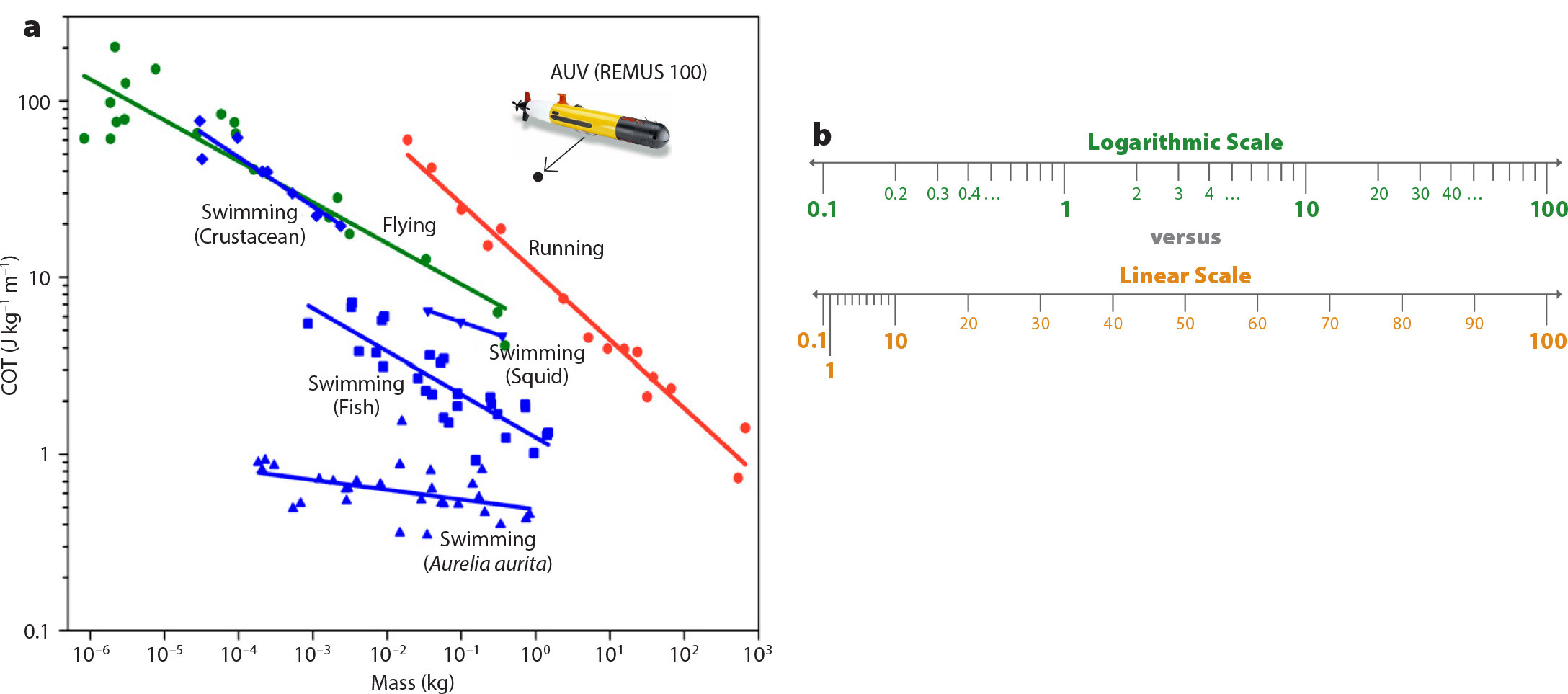
FIGURE 2. (a) Cost of transport (COT) versus mass for a variety of animals (colored by mode of locomotion, with swimming in blue, flying in green, and running in red) and a representative AUV (e.g., REMUS 100). Note that both axes are scaled logarithmically, and as illustrated, Aurelia aurita jellyfish possess the lowest COT values as the most energy efficient animals. Adapted from Gemmell et al. (2013) (b) A schematic scale showing the difference between a logarithmic scale (green) versus a linear scale (orange), to highlight the disparity in the COT values in the top logarithmic plot. Adapted from Lisa C. Muth, Datawrapper. > High res figure
|
This potential is most striking for jellyfish, organisms with the lowest known COT of all metazoans (Gemmell et al., 2013). Therefore, our initial challenge is to understand the dynamics of jellyfish swimming, with the goal of incorporating those design principles into engineered vehicles. Success could enable scaling of ocean sensors to swarms of millions, circumventing the energetic constraints that present a bottleneck for scaling existing engineered vehicles for whole-ocean exploration.
Uncovering the Hydrodynamics of Jellyfish Swimming
Jellyfish present unique challenges for the study of their swimming biomechanics. In many cases, the gelatinous organisms are too fragile to capture and bring into a laboratory environment (a limitation that further highlights the sampling bias of some ship-based measurement techniques). In addition, experiments in circulating laboratory water tunnels, known as pseudokreisels, designed with rounded corners and constant flow conditions, may not replicate the hydrodynamic stimuli that jellyfish encounter in their natural habitats (Purcell et al., 2013).
To address these challenges, we collaborated with colleagues to develop new in situ flow measurement techniques. By illuminating suspended particulates in the water column with lasers, and tracking particulate motion using high-speed videography, the new technique—a self-contained underwater velocimetry apparatus, or SCUVA (Katija and Dabiri, 2008; Katija et al., 2011)—enables divers to record the fluid dynamics of jellyfish swimming in the ocean (Figure 3).
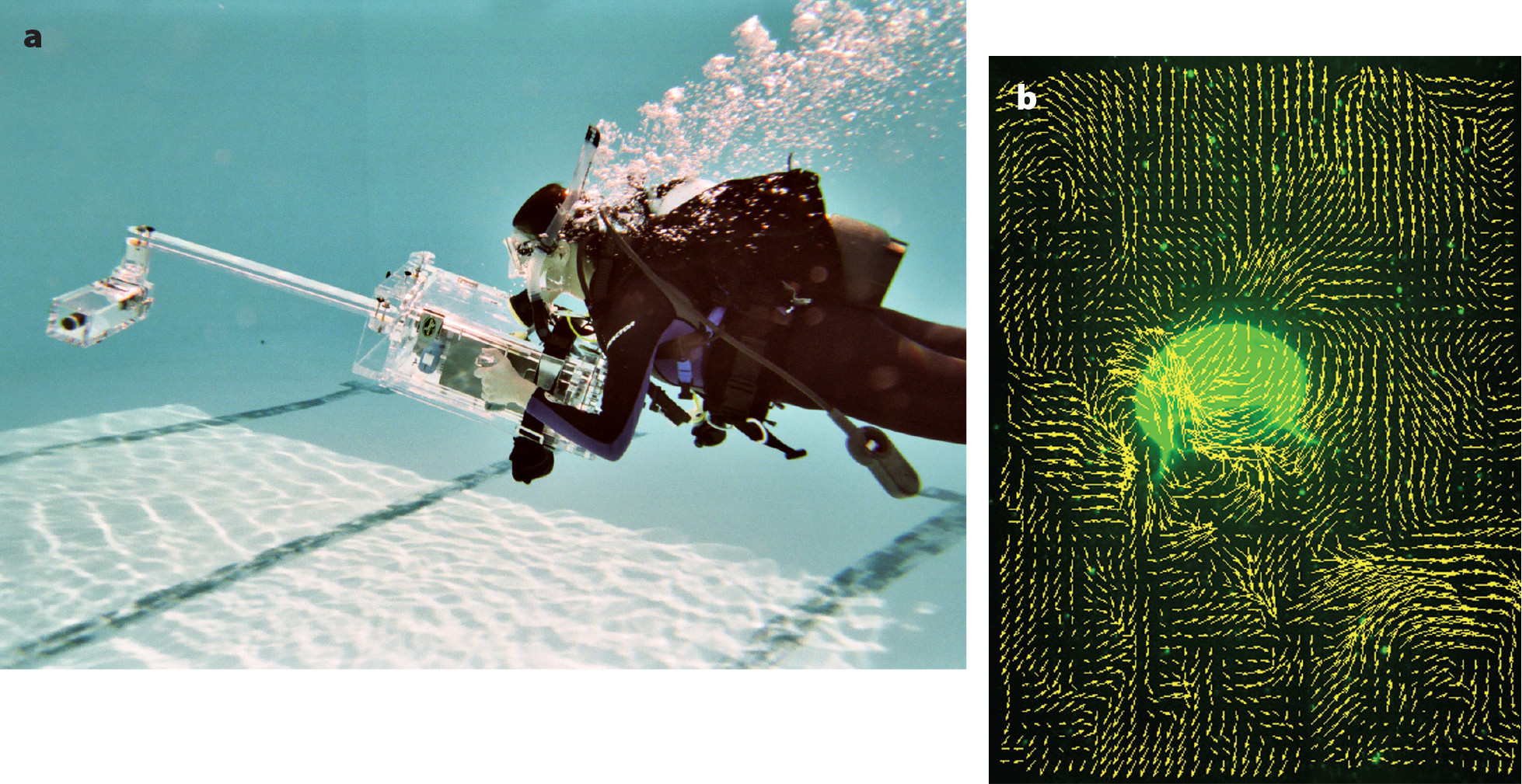
FIGURE 3. (a) Working in a swimming pool as a demonstration before deployment, a diver operates a self-contained underwater velocimetry apparatus (SCUVA), comprising a high-speed camera connected by a retractable arm to a laser source that allows visualization of the flow fields around animals in their natural habitats. (b) An example image of a measured velocity field (yellow vectors) around an Aurelia jellyfish swimming in Long Beach, California, demonstrates the capabilities of SCUVA. > High res figure
|
This new, quantitative perspective revealed three key mechanisms that contribute to the high energetic efficiency of jellyfish swimming. First, the periodic contraction and relaxation of the umbrella-shaped jellyfish body or bell creates vortex rings, toroidal (i.e., donut-shaped) swirling water currents, in an animal’s wake. During the contraction phase of swimming, the jellyfish bell activates a monolayer of muscle, oriented in a ring on the subumbrellar surface, to eject the volume of water under the body’s cavity, resulting in the shedding of “starting” vortex rings. These starting vortices entrain surrounding water as they grow, enabling the animals to transfer momentum to the fluid (i.e., generate thrust) with less energy expenditure than that associated with the conventional jet propulsion of a rocket (Dabiri, 2009).
Second, during the relaxation phase of swimming when the animal recovers to its resting body shape and water refills the subumbrellar volume, a second vortex ring of opposite rotational sense is formed upstream of the starting vortex shed. The formation of this “stopping” vortex, enhanced by the bell refilling phase, generates a secondary water current behind the animal that continues to push it forward even as it recovers from its initial swimming contraction. In fact, although starting vortices exhibit peak velocities during the contraction phase, the maximum circulation of stopping vortices can exceed the circulation of starting vortices, which underscores the influence of the stopping vortex over the entire swim cycle. Furthermore, the formation of this stopping vortex draws on the stored strain energy of the mesoglea (i.e., the gelatinous material that forms the majority of the jellyfish bell) via elastic tissue properties. Therefore, this process of passive energy recapture during the passive phase of jellyfish swimming allows the animals to swim 30% farther per cycle without additional energy input from the muscles. This contributes a 48% improvement in the COT for jellyfish (Gemmell et al., 2013).
Finally, using the water velocity measurements to infer the corresponding water pressure (Dabiri et al., 2014), we discovered that subtle body bending during swimming generates large anterior regions of low pressure on the animal body. Using minute radial muscle contractions at the bell margin to enhance bending, the animals are effectively “sucked” forward into low-pressure regions toward the exumbrellar surface, thereby further reducing their energy cost (Gemmell et al., 2015). Together, these three physical mechanisms contribute to the highly efficient locomotion of jellyfish (Costello et al., 2021). The challenge that remains is to leverage this new knowledge for the design of bio-inspired propulsion technologies.
Replicating Jellyfish Swimming Performance
Given our improved scientific understanding of the mechanisms leading to efficient jellyfish locomotion, the engineering charge is to design an underwater system that exploits those physical phenomena. A natural temptation is to simply mimic the shape and swimming kinematics of real jellyfish via robotic analogs. However, as yet, no engineered materials exhibit the same actuation and flexibility of the animal tissue, and even materials used in soft robotics—such as EcoFlex (Young’s modulus of 0.1 MPa), Sylgard (1.3–3.0 MPa), and gelatin-glycerol mixes (0.7–2.7 MPa) (Shintake et al., 2017)—are orders of magnitude stiffer than the elastic properties of jellyfish mesoglea (Young’s modulus of 340 Pa in one species of hydromedusae; Megill et al., 2005). Efforts to approximate jellyfish locomotion using existing engineering materials have successfully replicated their swimming motions, but with significantly higher energy costs of transport (Villanueva et al., 2011; Gemmell et al., 2013; Frame et al., 2018).
Our initial efforts focused on a strategy that leveraged existing propeller-driven vehicle platforms; one example of a modified vehicle design is shown in Figure 4. The design concept aimed to combine the high mechanical efficiency of the propeller with the high hydrodynamic efficiency of vortex ring formation. Specifically, the flow inlets to a shrouded propeller were designed to periodically open and close during operation. When toggled at high frequency, this periodic throttling generated vortex rings in the wake similar to those created by swimming jellyfish (Figure 4).
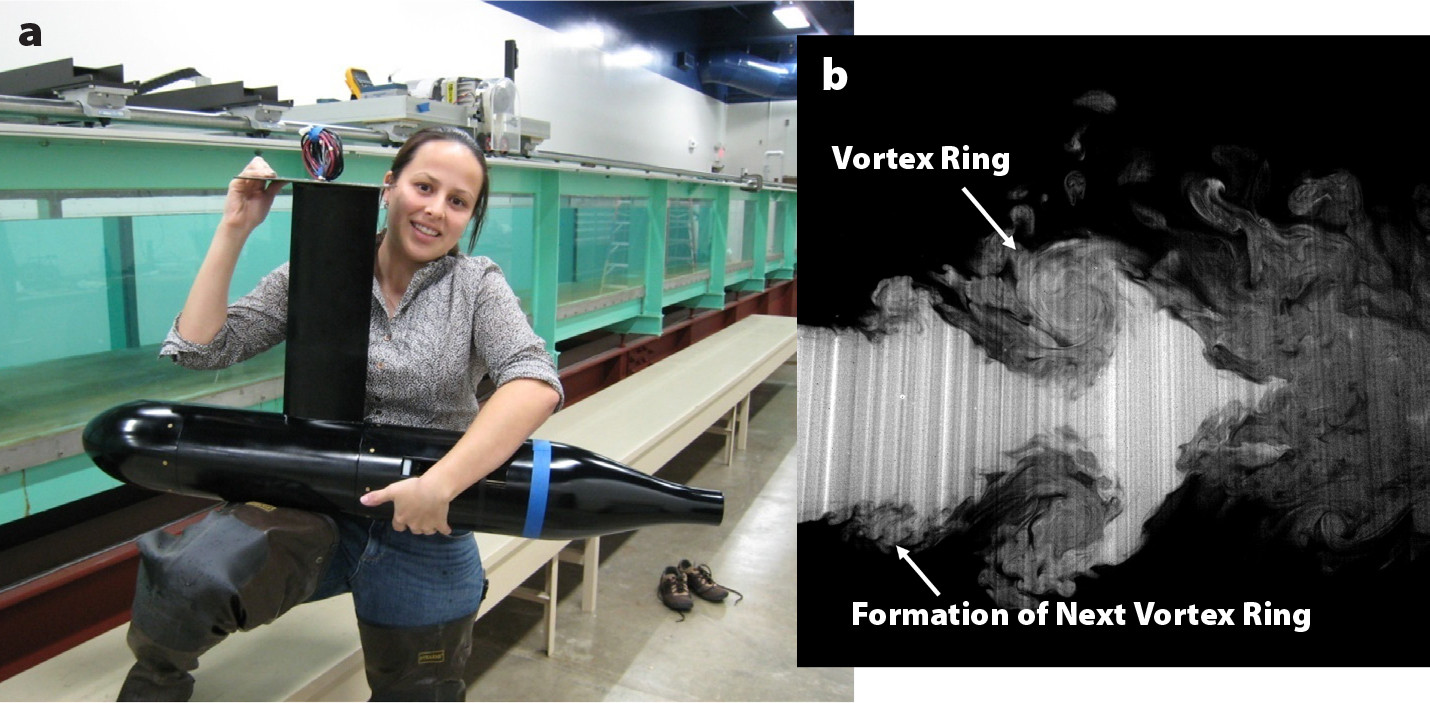
FIGURE 4. (a) Lateral flow inlets that lead to a shrouded propeller in this bio-inspired underwater vehicle periodically open and close to generate vortices. (b) A dye visualization of the vehicle wake reveals vortex ring formations. Flow is left to right. From Ruiz et al. (2011). > High res figure
|
Measurements of the vehicle’s performance in a 40 m long water channel demonstrated significant increases in hydrodynamic efficiency at all speeds tested, from 10 cm to 60 cm per second. In an intermediate range of speeds, the increases approached 40% over the efficiency of baseline, steady propeller operation (Ruiz et al., 2011). However, this increased hydrodynamic performance was often offset by reduced mechanical efficiency. In other words, the additional energy lost in the mechanism that created unsteadiness could exceed the hydrodynamic benefits from vortex ring formation.
Recognizing the limitations of engineering actuators, we subsequently explored the feasibility of using biological actuators—live muscle cells—to power the engineered swimming robots, in much the same way that jellyfish use their own muscles to swim. Live muscle has the advantage of being multifunctional. The tissue provides the necessary actuation to set the surrounding water into motion. It also provides structural scaffolding, and it can heal itself if damaged. In addition, unlike engineered, mechanical systems, the muscle tissue provides chemical energy storage, and it facilitates direct chemical energy conversion, as opposed to indirect conversion from a battery to a motor, and then through gearing to the ultimate body motion. This work leveraged muscular thin film technology pioneered by collaborators at Harvard University that is based on cardiomyocytes (i.e., cardiac cells) extracted from rats (Feinberg et al., 2007).
By leveraging these recently developed tissue engineering techniques, rat cardiac cells were seeded onto silicon platforms and driven by external electric fields to incite their contractile motion. As shown in Figure 5, it was possible to replicate the details of the muscle architecture down to the micron scale. The resulting bio-inspired swimmers proved effective at replicating natural animal swimming motion and fluid dynamics (Nawroth et al., 2012). While this work demonstrated a paradigm of bio-hybrid robots that has subsequently been adopted for other swimming and crawling organisms (i.e., a biohybrid fish; Lee et al., 2022), its use for ocean exploration is limited in practice by the live tissue, which only remains viable in specific aqueous media (i.e., Tyrode’s solution). Hence, the bio-hybrid robotic swimmers are limited to laboratory environments. Additionally, the scale of these swimmers is currently limited to approximately one centimeter in diameter, and scaling the size upward could be nontrivial. Nonetheless, these bio-hybrid tissue engineered constructs provide a valuable platform for studying efficient bio-inspired locomotion and provide further insight into bioengineering techniques for medical applications, such as the potential for seeding human cardiomyocytes in more complex, bio-compatible scaffolding structures to grow artificial organs for transplant patients, among other ambitious goals. Moreover, the knowledge gained from these studies led to our ultimate strategy to expand the toolset of bio-inspired ocean exploration.
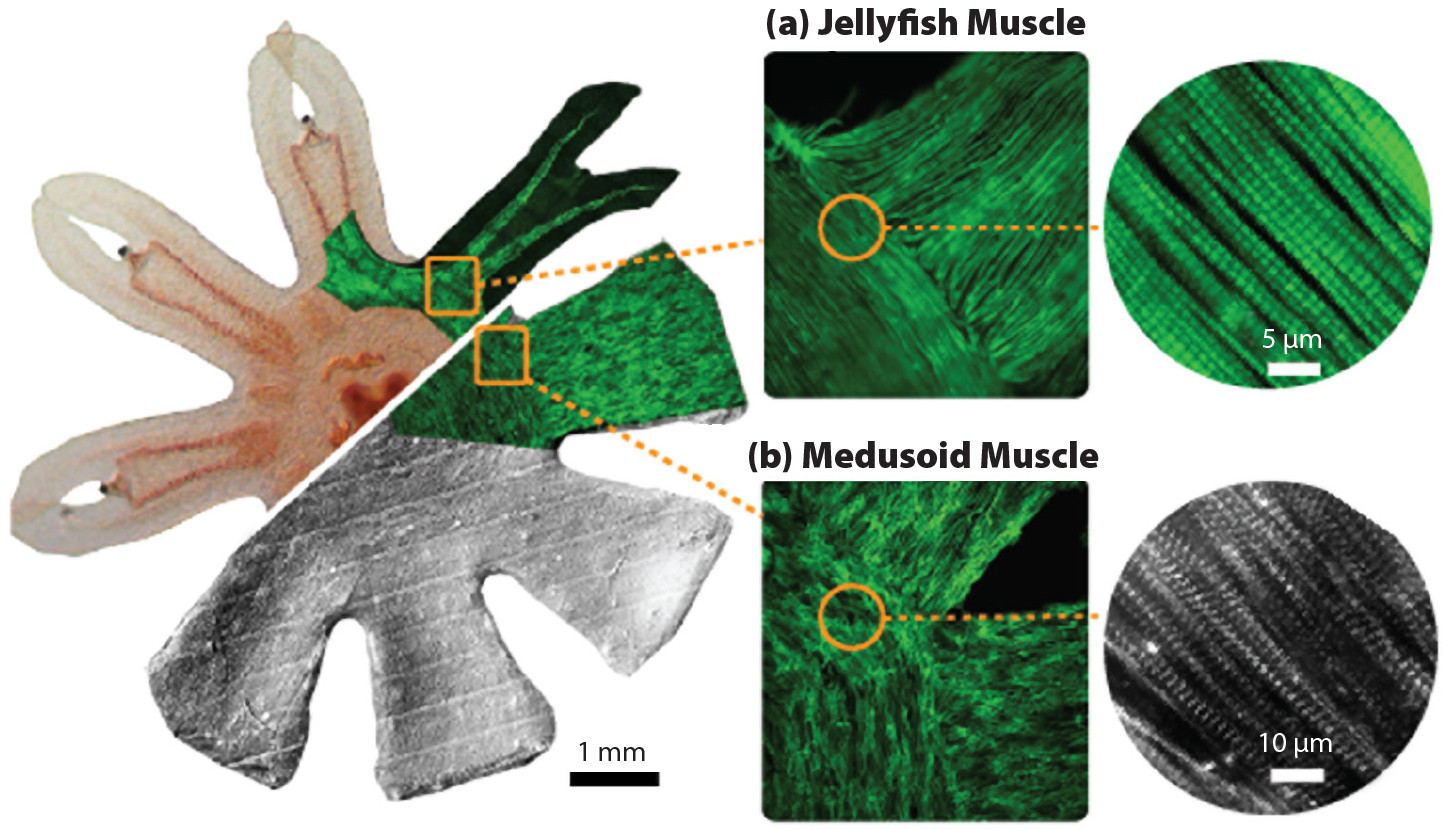
FIGURE 5. Multi-scale comparison of the muscle architecture of (a) a real jellyfish and (b) a bio-inspired, tissue engineering construct. From Nawroth et al. (2012). > High res figure
|
Remotely Controlled Jellyfish
Our scientific journey led us to the realization that global ocean exploration might be most effectively achieved by jellyfish themselves. This strategy takes advantage of the efficient hydrodynamics and actuation that already occur naturally in the ocean. Jellyfish are self-powered by feeding on prey within the water column. They are naturally self-healing. They exhibit neither latitude nor depth limitations in the ocean, even thriving in hypoxic regions and climate change induced extreme conditions to which many other species cannot adapt. Their performance at depth is especially important, given that we already have an excellent set of tools for studying the upper ocean (Figure 1). The pressure of the deep ocean, combined with our inability to probe it from the surface using most traditional technologies, has made that part of the ocean nearly impenetrable for prolonged temporal observations at any spatial scale. In part because they do not possess a swim bladder, jellyfish can be found as deep as the Marianas Trench, at least 3,700 m below the ocean surface (Brooke et al., 2017). While accompanying sensors would still require hardening to endure pressures at those depths, that task can be more tractable than hardening an entire AUV to withstand the high pressure in the deep ocean while also remaining sufficiently small as to function with an efficient propulsion system. Finally, the use of bio-inspired robotics using animals, with their more natural acoustic and wake signatures, can potentially allow for closer studies of the flora and fauna in environmentally sensitive regions that might otherwise be impacted by traditional vehicular noise signatures. Thus, one approach for ocean monitoring is to use remotely controlled jellyfish, or bio-hybrid robotic jellyfish. But to address the integration of a robotic system onto live jellyfish, we must first consider several ethical and logistical questions.
Ethical Considerations
It is important to address the ethics of this approach preemptively. To augment these animals, we aim to “steer” the jellyfish using electronics, analogous to the control of farm animals for agriculture. To be sure, even the concept of electronic stimulation of the neuromuscular system has been well established. Such stimulation is the core of artificial cardiac pacemakers, transcutaneous electrical nerve stimulation (TENS), dry needling, and other forms of physical therapies for medical treatment and intervention with minimal pain or discomfort to the patient. Robotic stimulation of nerves and muscles can therefore apply more broadly to different species of jellyfish, aquatic invertebrates, and other marine animals (with further consideration toward animal ethics).
Such ethical considerations are not trivial, even among higher order invertebrates (De Waal and Andrews, 2022). In the case of jellyfish, a lower order invertebrate with distributed nerve nets, we note their lack of a central nervous system and of nociceptors, or pain receptors, which makes them good candidates as subjects for animal research. Although pain is a subjective experience, nociception is an objective physiological response to stimuli (Sneddon, 2018). Because jellyfish do not have either central nervous systems or nociceptors, other proxies can be used instead, in line with the precautionary principle, to minimize even potential harm or distress to subjects (Birch, 2017). While the species of jellyfish used in this research, Aurelia aurita, can exhibit indicators of stress under some environmental stimuli (e.g., excretion of mucus), no such response has been observed using the techniques implemented presently. Furthermore, once the swim controllers were removed from the animals, their behaviors returned to baseline conditions, including swimming, feeding, and reproducing in the laboratory. Reproduction, in particular, can be negatively impacted by compounding stressors, and producing offspring even throughout laboratory tests is an indicator of animal well-being.
In addition to the ethical considerations of the individually augmented animals, we also consider the broader environmental ethics of deploying bio-hybrid robotic jellyfish in ocean environments. First, care should be taken to ensure that the jellyfish species used in specific regions of the ocean are endemic so they do not become invasive species. To be sure, many jellyfish species, both endemic and invasive, have become more problematic because of their increased survivability under changing environmental factors, such as increased ocean temperatures and acidities, compared to other metazoans in the ecosystem. To counter these effects, jellyfish should be captured, instrumented, and released only from their natural environments. Moreover, future research enabled by the remote control of jellyfish could be used to better understand the origin of high evolutionary fitness in jellyfish and their robust survival tactics.
For our field experiments, we used species endemic to the region and closely monitored the individual animals to prevent them from escaping or coming to harm, as well as to ensure that no plastic or electronic byproducts were discarded in the ocean. For an expanded future research program, an important consideration is the potential introduction of waste into the ocean from deployment of large numbers of bio-hybrid robotic jellyfish. Because jellyfish are consumed by some natural predators, it is feasible that a predator could incidentally ingest a robotically stimulated jellyfish that has been deployed for more remote or autonomous ocean expeditions, where they remain unmonitored or where human monitors are unable to intervene. For ethical purposes, the environmental impacts can be minimized by using more natural or biodegradable materials for the electronic components, sensors, and housing elements (Irimia-Vladu et al., 2012). For scientific purposes, the use of multiple jellyfish as swarms to plan for data loss is key, although real-time data collection methods would be ideal to obtain sensor information before the jellyfish are offline.
These ethical considerations will be continuously revisited as the work progresses and becomes more imminent. We have collaborated with bioethicists to ensure that we apply a well-informed framework to considerations of any new research in this area, with attention toward individual animals, jellyfish as a species, and potential environmental and ecological effects (Xu et al., 2022).
Robotic Implementation
For robotic control, the choice of using this species of jellyfish goes beyond the simplicity of their bodies and nervous systems for ethical considerations. Aurelia aurita, or moon jellyfish, the most common species of jellyfish, are found in a wide range of natural environments. Furthermore, they are the most widely studied jellyfish species, and they offer practical advantages for laboratory experiments because of their ease of care in artificial environments and lack of harm to humans. Although they do possess nematocysts, their stinging cells cannot penetrate human skin. Furthermore, the jellyfish used in this research can be obtained from those bred in captivity, where generations of breeding and a lack of competition for food have further decreased the capabilities of their stingers to inflict injury to human handlers.
To control jellyfish swimming, small microelectronics are embedded in the body tissue. The microcontroller and battery system are placed in the center of the bell where there is most tissue mass, and electrodes are located near the bell margin to innervate the muscle ring directly (Figure 6). The components necessary for a complete system are commercially available at very low cost; a control module can be assembled for a few dollars, with decreasing costs as the process scales toward mass production. This cost advantage can enable the present approach to scale to millions of devices, as the animals themselves can be mass-produced by asexual reproduction for only minimal animal care costs. Regarding human labor to insert the robotic system into the animal, the insertion process is also quick, requiring less than a few seconds per animal. Thus, the key question is to assess the swimming performance of the robotically controlled jellyfish.
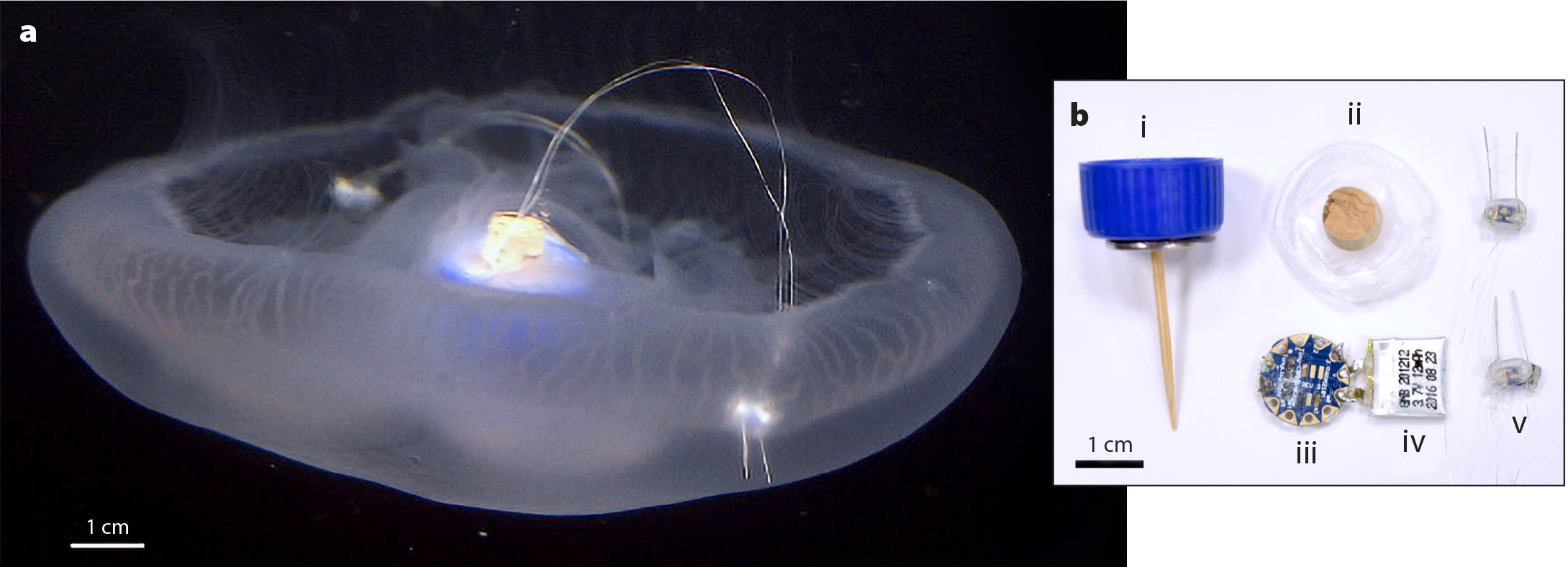
FIGURE 6. (a) Photo of Aurelia jellyfish with electrodes implanted at its margins and a microcontroller located at its center. (b) Swim controller components: (i) waterproof housing and attachment pin, (ii) plastic film sealant, (iii) TinyLily miniprocessor, (iv) lithium polymer battery, and (v) platinum electrodes with LED indicators. From Xu and Dabiri (2020). > High res figure
|
Figure 7 compares the swimming speed of the externally controlled jellies to that of jellyfish swimming under endogenous control, without the influence of the swim controller (but with the swim controller implanted), under laboratory conditions in the absence of flow. The robotically controlled jellies swim up to three times faster than natural jellyfish. We attribute this to the more regular swimming of the externally controlled jellies, as well as the fact that they can be induced to contract at faster frequencies than they exhibit naturally. Hydrodynamic models also indicate that specific jellyfish morphologies (such as more prolate bell shapes) can be chosen to obtain more enhanced swimming speeds for future robotic control.
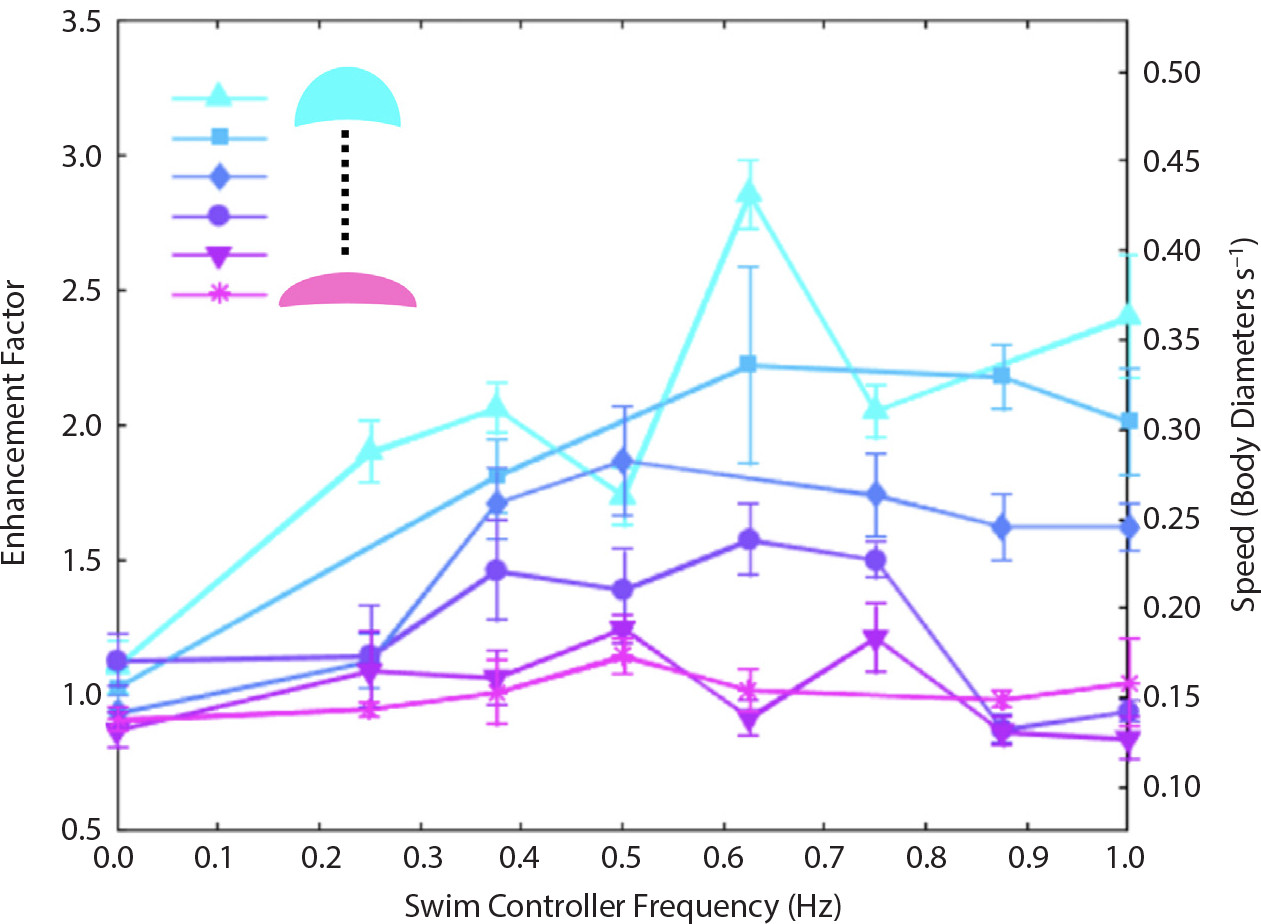
FIGURE 7. Swimming speeds and enhancement factors for swim controller frequencies up to 1 Hz. Each animal studied is represented by a different color curve, and the size range per animal reflects changes in bell growth over time (experiments were conducted over several days). Normalized speeds (body diameters per second) are indicated on the right ordinate axis. The enhancement factor is defined as the normalized swimming speed scaled by the mean of the normalized 0 Hz speed (i.e., in the absence of stimulation, in which the swim controller is embedded but inactive). From Xu and Dabiri (2020). > High res figure
|
Interestingly, these externally driven jellyfish were also found to swim with better energy efficiency as well, as measured by their metabolic requirements and the COT metric. Specifically, the bio-hybrid jellyfish robots were observed to swim up to three times faster than their natural counterparts at only twice the energetic cost to the animal. For the same swimming efficiency as the natural counterparts, the energetic cost of swimming at triple the speed would correspond to a nine-fold increase in COT. Hence, robotic control revealed latent swimming performance that the jellies do not exhibit naturally. We can reconcile these observations by noting that this species of jellyfish is primarily a filter feeder. Its swimming is secondary to the function of drawing surrounding water (and suspended prey) toward its feeding appendages. Neither swimming speed nor swimming efficiency is necessarily a priority for these organisms in nature, compared to survivability of the individual and evolutionary fitness of the species. However, for our engineering applications, those characteristics can potentially be exploited to achieve the goal of global ocean exploration. Indeed, the external power consumption of these bio-hybrid robotic jellyfish is 10 to 1,000 times less than that of existing underwater robots, including bio-inspired robots and more traditional underwater vehicles (Xu and Dabiri, 2020). Initial field trials of the robotically controlled jellyfish have also demonstrated that the system described here can be deployed in the real ocean, with similar enhanced swimming speeds as observed in the laboratory (see Figure 8c,d for representative images of the setup; Xu et al., 2020).
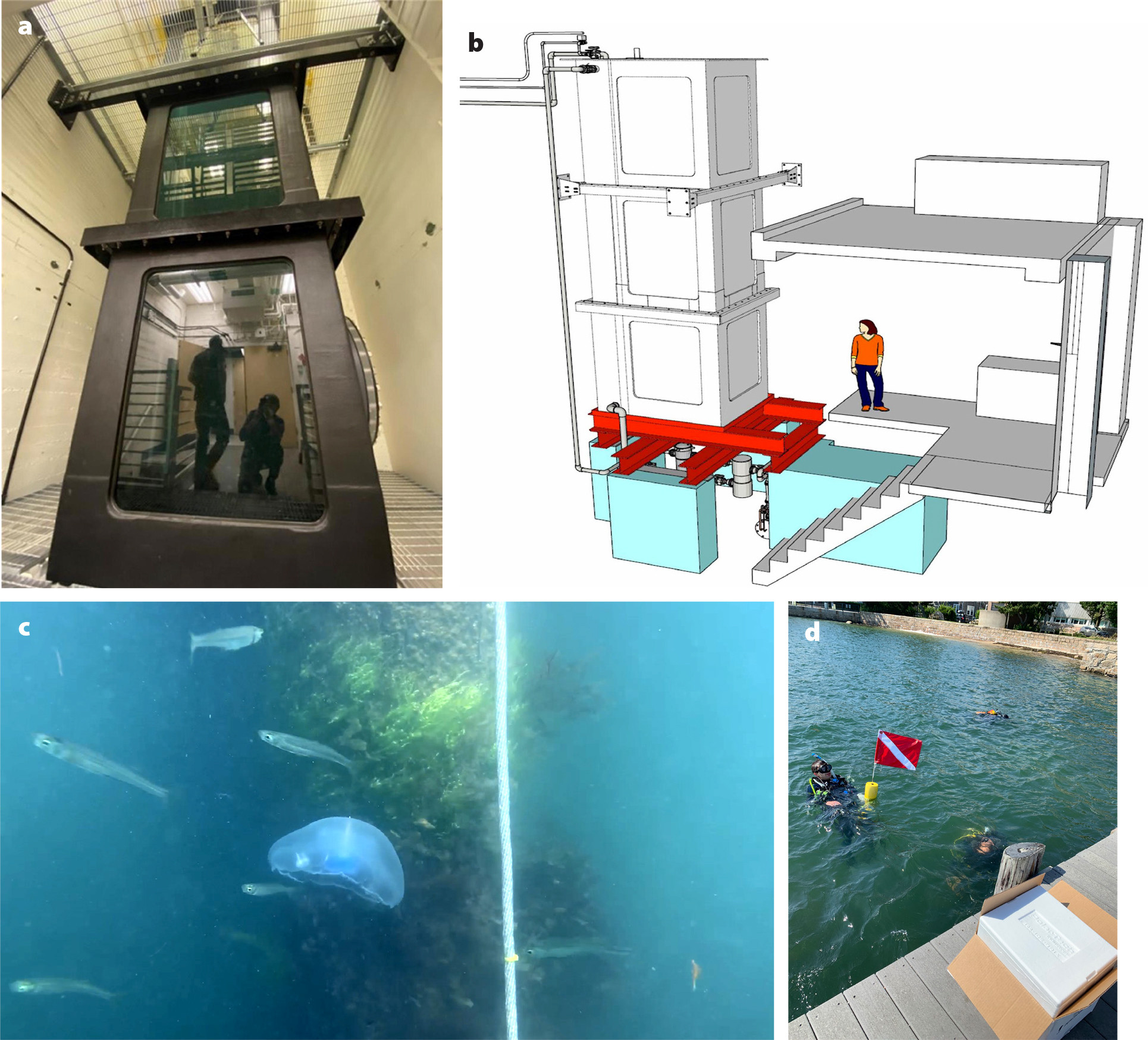
FIGURE 8. Test environments for bio-hybrid robotic jellyfish swimming. (a) Laboratory tank (20 ft height × 5 ft width/length, or 6 m height × 1.5 width/length) with controllable upwelling and downwelling water currents for testing jellyfish and bio-robotic swimming in controlled systems. (b) Schematic illustration of the tank to scale. Representative images of (c) a robotically controlled jellyfish swimming in an ocean environment, monitored by (d) scientific scuba divers off the coast of Woods Hole, Massachusetts. From Xu et al. (2020). > High res figure
|
The Future of Bio-inspired Ocean Exploration
Although initial field tests yielded promising results, several additional developments are required to create a viable ocean sensor. First, the robotic control is currently limited to one-dimensional steering in the direction of the current jellyfish heading. Full six degree-of-freedom steering of the jellyfish will likely require more complex muscle stimulation strategies than that implemented to date (Hoover et al., 2021), such as using various asymmetric activation patterns of electrodes on the swim muscle. Asymmetric activation patterns of electrodes can be used to initiate bell pitching due to torque, such as by stimulating one electrode to initiate time-dependent muscle contractions that travel in a bidirectional wave from the point of electrode contact. Potential control over finer muscle near the bell margin for increased turning maneuvers could also be used to mimic the animals’ natural turning enhancements.
In addition, the existing control system is open loop (i.e., the control is not contingent on the current state of the jellyfish, such as its heading or sensed environment). We are excited about the potential of common robotics platforms such as Arduino, which can achieve closed-loop control with limited additional system complexity. The goal is for eventual robotic swarms to behave autonomously. Applications of emerging techniques in artificial intelligence such as reinforcement learning (Gunnarson et al., 2021) can enable robust control with limited onboard computational processing, and physical tests can be performed in a three-story tall tank with controllable upwelling and downwelling water currents at the California Institute of Technology (Figure 8a,b).
The utility of the jellyfish sensors ultimately depends on their measurement capabilities. Indeed, the comparison with AUVs is tenuous, given the AUV’s capability for carrying a much larger payload individually than jellyfish. Groups of robotic jellyfish operating in concert might be able to match functions typically achieved by single, larger systems. Nonetheless, an important next step is to demonstrate ocean-relevant measurements using the robotic jellyfish systems. Vertical profiles of ocean physics, chemistry, and biology used for studying temporal and spatial patterns, such as carbon sequestration and nutrient transport, are a promising first application area. Such measurements could be a powerful complement to the physical and biogeochemical measurements of the Argo and BGC-Argo projects, and reduce the costs needed to obtain data at higher resolutions to study the biogeochemistry of the ocean on a worldwide scale. Large swarms of jellyfish robots also have the potential to improve predictions of ocean climate models. Miniaturized cameras could also be used to observe regions of interest in the ocean that are currently unexplored, such as near sensitive coral reefs or in deep-sea trenches where taxonomic and animal behavioral data is sparse.
We can also take advantage of this bio-inspired system for its innate abilities and physiology. For instance, future projects might use the jellyfish’s innate sensory information to translate the biological signals received from their sensory organs, or rhopalia, into information that can be used for more autonomous behaviors. In addition, the metabolic systems of jellyfish provide considerable untapped potential—new advances could allow these animals to power their own onboard electronics through chemical conversion processes.
Practical implementation of this technology could be limited by the longevity of jellyfish functioning, so it is important to characterize that limit. Desensitization of robotically stimulated jellyfish muscle, which could render external control ineffective, has not yet been observed. To date, laboratory experiments have been conducted over a maximum two-day period to allow the animals to rest and recover. Although successful muscle stimulation appeared consistent during these 48-hour periods, longer-term experiments should be conducted in controlled laboratory environments to determine whether there is observed muscle fatigue, changes in the strength of muscle pulses, and subsequent changes in swimming speeds. These tests can also quantify whether the filter feeding capabilities of jellyfish are impacted by robotic control, and if the quantity of food sources available in the water can sustain the additional metabolic costs of faster swimming speeds. Additionally, it is unclear whether jellyfish that die while deployed in the ocean would be a hindrance to data collection. It might be possible to stimulate their swimming muscle for a period post-mortem, as has been demonstrated recently in arachnids with the concept of “necrobotics” (i.e., using robotic systems to stimulate dead organisms; Yap et al., 2022). As noted above, ethical considerations must precede pursuit of these approaches.
Even with ideal results regarding the longevity of individual jellyfish, because the ocean is a complex, unstructured environment for any robotic system, the longevity of individual robotically controlled jellyfish is unknown due to natural predation. To ensure that lost systems do not contribute to ocean pollution or cause harm to other organisms, advances in biodegradable electronics (Irimia-Vladu et al., 2012; Li et al., 2018), and either natural materials or biodegradable housing and structural elements, should be pursued to replace conventional hardware in these systems. Further ethical consideration should be given to addressing any concerns, from the individual level for jellyfish test subjects (e.g., by continuous monitoring of stress-related molecular biomarkers) to species-wide and ecological levels (e.g., by considering potential unintended consequences), so that this new approach is a model for both its technological innovation and its ethical pursuit of research.
Recovery of measurements from the robotic jellyfish faces the same challenges encountered when using existing AUVs. Initial efforts are focused on surface recovery. Rendezvous with complementary systems in Figure 1 could provide another strategy for efficient data recovery. Bio-hybrid robotic jellyfish can and should be used synergistically with these existing technologies in order to complement the strengths and weaknesses of each system. For example, to address the issue of bio-hybrid robotic jellyfish whose swimming speeds (on the order of centimeters per second) are slower than those of traditional underwater vehicles (on the order of meters per second), expeditions could use AUVs to tow jellyfish swarms to regions of interest and release them to disperse for data collection. This approach could also be useful for hosting a recharging station for the jellyfish robots on the AUV, or for using existing short-range underwater communication technologies. Finally, because jellyfish are zooplankton, or metazoans primarily carried by currents in the ocean, we can potentially use robotic jellyfish as Lagrangian sensors in areas with strong currents, such as the East Australian Current, to disperse these swarms over a larger spatial scale.
Indeed, the use of bio-hybrid robotic jellyfish need not exist as a stand-alone tool for the entire future of ocean observation. Many of the most pressing global issues or scientifically challenging problems are at the intersection of multiple disciplines. The application of remotely controlled jellyfish provides an exciting prospect for such interdisciplinary work. Much like we cannot exist as individuals in this world without communities, the global collaborative efforts and challenges of the UN Ocean Decade highlight how synergistic efforts can be used to better understand the ocean and effects of climate change.
Success in this endeavor could enable us to revisit scientific questions that have lain dormant in recent years due to our inability to access the ocean at reasonable costs. The prospect of biogenic ocean mixing in diurnal vertical migrations is an exciting example (Katija and Dabiri, 2009; Houghton et al., 2018). More generally, the low cost of this technology has the potential to democratize ocean exploration and benefit areas that rely more heavily on the ocean economy, including disproportionate numbers of developing nations. The current high cost of ship time, on the order of 20,000 USD to 50,000 USD per day, creates a structural barrier to wider participation in ocean science. While the high cost has the ancillary benefit of promoting collaboration of larger teams in order to pool available resources, it can also lead to the exclusion of scientists not affiliated with those groups. More perniciously, it can prevent the exploration of new ideas that have not yet gained acceptance as the dominant paradigm in a field, as experimental ocean science is typically too expensive for a lone investigator testing an unorthodox hypothesis to pursue. Perhaps a solution lies in the similarly unorthodox approach summarized in this Revelle Lecture.
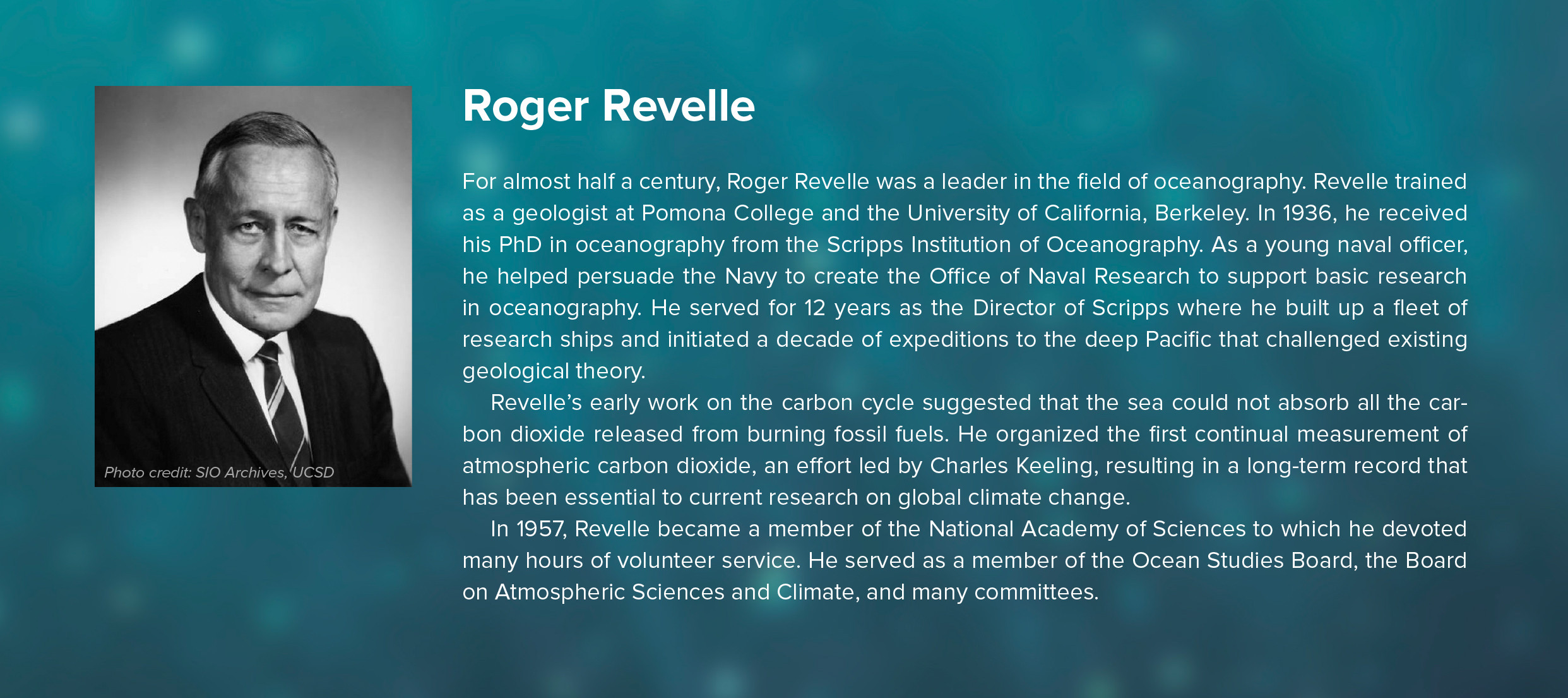
|
Acknowledgments
The authors gratefully acknowledge seminal contributions to this work from a diverse set of students and colleagues, including Kakani Katija, Lydia Ruiz, Robert Whittlesey, Janna Nawroth, Jack Costello, Sean Colin, Monty Graham, Brad Gemmell, Kelly Sutherland, and Kit Parker. Funding from the National Science Foundation, the Office of Naval Research, and the John D. and Catherine T. MacArthur Foundation was essential to conduct this research.