Introduction
Offshore wind energy development has been identified as a priority for both the US and European nations, but the actual impacts to human activities, marine wildlife, and habitats are not well understood. In the United States, much of this development occurs in Essential Fish Habitat1 for federally managed and commercially important fish species. In Europe, marine protected areas fall under the European Commission initiative Natura 2000, which provides guidance regarding how best to ensure that wind energy developments are compatible with conservation measures for key fishes and habitats (European Commission, 2010). Designing effective monitoring and mitigation programs to assess potential wind farm influences requires evaluation of impacts to taxa and communities. Baseline data and experiments are critical. Without them, it is impossible to test predicted impacts and effectively examine potential changes.
To date, most studies on the potential effects of offshore wind farms (OWFs) have tended to focus on the installation and operation phases. However, the four key phases of OWF development, each with different noise emissions, take place over a much broader timeline that encompasses: (1) prospecting and site surveys, (2) construction, (3) operation, and (4) decommissioning (Figure 1). All four phases produce sounds that have the potential to influence marine life, and each should be considered in its own context.
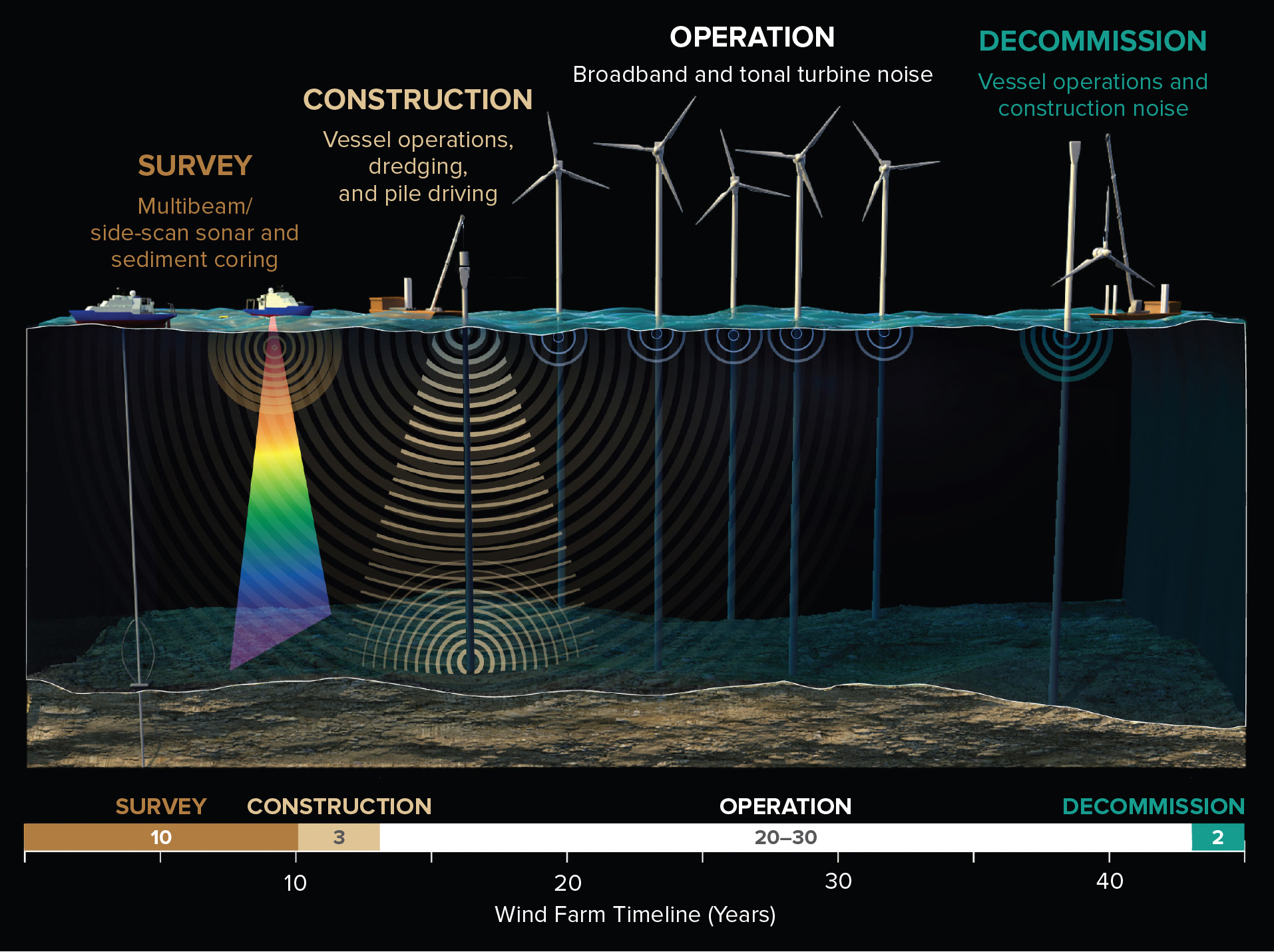
Figure 1. Acoustic life of an offshore wind farm area, including during site surveys, construction, operation, and decommission. > High res figure
|
One of the vital areas of potential effects, as identified by European and US agencies alike, is acoustic disturbance from “noise.” The term noise is often used to describe unwanted sounds that are considered to be unpleasant, loud, disruptive to hearing, or can otherwise disrupt behaviors, physiology, or detection of a particular signal (Popper and Hawkins, 2019). In some cases, the terms ambient noise or background noise may also be used to describe sound generated by natural sources, as well as by anthropogenic sources, especially where they may interfere with the detection of biologically relevant cues (Popper and Hawkins, 2012; Dooling and Blumenrath, 2016). Here, we use this broader, encompassing definition.
Noise impacts can generally be placed into often overlapping categories, including physical injury and physiological and behavioral influences (Figure 2; Hawkins et al., 2015). Briefly, intense, impulsive sounds and long-duration exposures can induce physical damage, such as auditory hair cell loss in fish and physiological stress responses. Anthropogenic noise can mask detection of biologically important signals used for communication, predator avoidance, and prey detection, and can influence behaviors. For example, animals may move out of a noise area, potentially disrupting foraging or breeding. While sound pressure level (dB re 1 µPa) is the most often quantified acoustic variable, sound can be described in many ways (see Box 1 for a short acoustics primer). Sound exposure level (SEL [dB re 1 µPa2 · s]) is used to accumulate the acoustic energy of an exposure over time, although for regulatory purposes this exposure duration is often calculated for (or limited to) 24 hours. Other parameters, such as acoustic particle motion, are particularly important for fishes and invertebrates, but such parameters are often overlooked or unquantified.
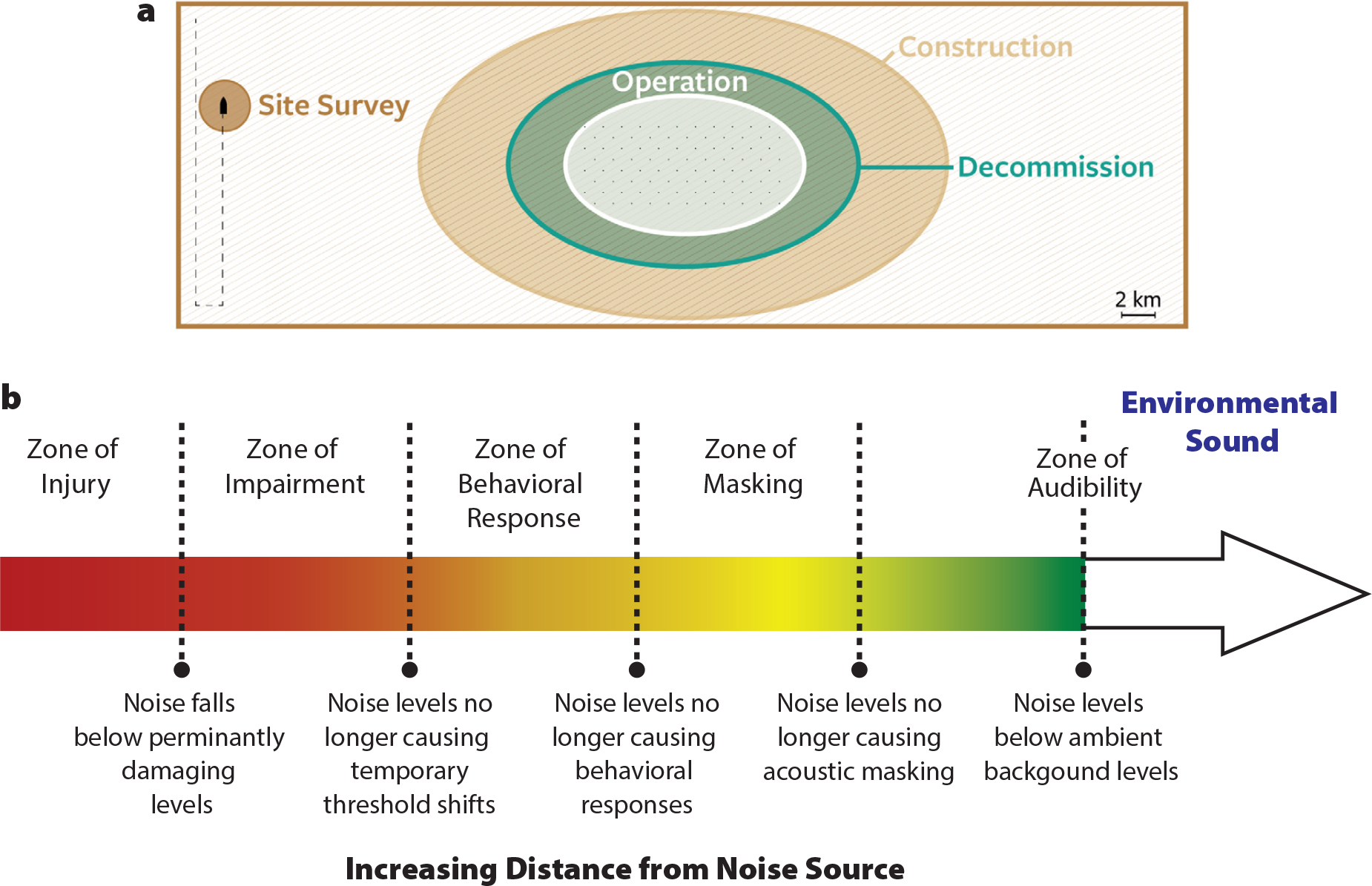
Figure 2. (a) Spatial representation of acoustic impacts drawn to a general scale. Note that site surveys tend to occur in somewhat standardized patterns (vertical lines toward the left, covering most of the wind farm lease area but temporally short). High-amplitude pile-driving construction noise can travel a substantial distance and may show impacts many kilometers from the source. Operational noise tends to be lower amplitude and extend over shorter ranges. Similar trends might be expected for decommissioning wind farms, although certain activities such as jet-cutting are high amplitude and would likely persist over long distances. (b) The potential effects of noise with distance from source. Generally, noise and impact on individual animals may be greater closer to the source. Effects change with increasing distance from the source, as the acoustic signals change including that received levels decrease. Figure modified from Dooling and Blumenrath (2013). > High res figure
|
Box 1. Acoustics Primer
Sound travels efficiently in the ocean at about 1,500 m s–1—five times faster than it does in air. Acoustic signals consist of two components: sound pressure and particle motion. The former is a compression and rarefaction wave. It is a scalar quantity that acts in all directions. It can be described in terms of its magnitude, as well as its temporal and frequency characteristics. In contrast, particle motion is a back-and-forth motion and, as such, is a vector quantity. Accordingly, particle motion can be described not only by specifying its magnitude and temporal and frequency characteristics but also by its direction of motion. Sound pressure is expressed in SI units of pascals (Pa) or micropascals (μPa). Particle motion may be expressed in terms of the particle displacement (SI unit: meter) or its time-related derivatives: particle velocity (meters per second) or particle acceleration (meters per second squared). Sound intensity is the product of the sound pressure and the particle velocity, for which the SI units are watts per meter squared.
In addressing acoustic impacts, it is fundamentally important to understand sensitivity. All fishes (including elasmobranchs) and an increasingly identified number of invertebrates detect and use particle motion, particularly at frequencies below several hundred hertz. Detection of pressure in water requires a compressible cavity such as an air bubble or swim bladder. Some fishes have evolved with air bubbles located just under external hair cells or with a variety of swim bladder extensions to the inner ears, all adaptations that enhance their detection of pressure. When addressing the effects of sounds on fishes and invertebrates, it is vital to describe the sounds in terms of particle motion as well as sound pressure. This may be done by measuring the particle motion directly or by conducting experiments under free-field acoustic conditions, where particle motion can be predicted from measurements of sound pressure. However, near boundaries, such as the seafloor and sea surface, or in shallow water, particle motion cannot easily be predicted by pressure so direct measurements are ideal.
Offshore Wind Farm Construction Phase
Modern offshore wind turbine generators (WTGs) are designed to be considerably larger than their terrestrial relatives, as it was generally recognized early that the aggressive environment and the need for reduction in maintenance due to higher costs incurred offshore would need specifically designed turbines. The largest wind turbine available to date is the 14 MW 14-222 DD model (222 m rotor diameter) launched by Siemens Gamesa, which overtakes the General Electric’s 12 MW Haliade-X platform released in 2018. However, currently the largest installed and operational turbine (as of autumn 2018) is an 8.4 MW tower at the European Offshore Wind Deployment Centre [EOWDC], (formerly, Aberdeen Offshore Wind Farm, UK), which will soon be superseded by the 9.5 MW turbines installed at Triton Knoll, where with the first foundations were installed in January 2020.
Types of Foundations
Monopile Foundations
The monopile is the most common type of wind turbine foundation. It was used for 70%–80% of all offshore wind turbines that were operational in 2016 in Europe and 100% in the United States (Díaz and Guedes Soares, 2020; Wind Europe, 2017). This foundation primarily consists of a cylindrical steel structure driven 18–45 m into the seabed using hydraulic or diesel hammers that employ impact or vibratory/percussive pile driving (Energinet, 2015; Andersson et al., 2016).
Jacket and Tripod Foundations
A jacket foundation usually consists of a cross-braced steel frame with three of four supporting piles or “legs” driven 30 m to 60 m into the seabed using similar pile-drive methods as for the monopiles (Energinet, 2015). Alternatively, the jacket is secured to the seafloor via slimmer piles that are driven through “sleeves” or guides mounted to the base of each leg of the jacket structure. Jackets accounted for ~12% of the foundations installed in Europe in 2016 and are likely be used in installations of WTGs in deeper locations (25–50 m; Wind Europe, 2017; Díaz and Guedes Soares, 2020).
Gravity-Based Structures and Suction Buckets
Other types of foundations and substructures are also used in offshore wind farms, though gravity-based structures and suction buckets are less utilized than the monopiles or jackets. These others are deemed more “eco-friendly” alternatives, as they do not involve large periods of percussive pile driving. Their foundations sit on top of the seafloor or at relatively shallow depths and greatly reduce the amount of vibration, noise, and suspended sediment generated in the construction phase (Oh et al., 2018).
A gravity-based structure generally consists of a large circular pile with a concrete base (sand, rock, and iron) held in place by gravity. These structures are usually used for smaller WTGs that range from 450 kW to 6 MW. They are suitable for firm seafloor conditions and are often used in areas of relatively large ice loads (Díaz and Guedes Soares, 2020). For installation, a suction bucket or suction caisson that consists of an inverted bucket-like structure is placed at the desired position, and the water trapped inside is pumped out. This creates suction inside the base that, combined with the pressure of the overlying water, forces the structure to penetrate the seabed, though not to the same extent as monopile or jacket foundations. This foundation is best suited for deeper waters (>50 m) and large WTGs (Oh et al., 2018; Díaz and Guedes Soares, 2020).
During installation, significant large and small vessel support is required, typically involving one or more construction vessels and a number of smaller support and personnel vessels. Construction vessels may include jack-up or semi-jack-up vessels or ships capable of dynamic positioning and deploying equipment such as cranes and hydraulic hammers (Energinet, 2015; Miller et al., 2018).
|
Here, we seek to provide a brief review of the acoustic effects associated with OWFs on fish and invertebrates with an emphasis on fisheries resources, although there are a plethora of fish and invertebrate taxa that are important fisheries resources but that have not received much attention with respect to noise. Thus, this is a general summary of current understanding, not an all-encompassing review. We include all four phases of an OWF’s lifetime (Figure 1), as each may have detrimental effects.
1 Essential Fish Habitat is defined by the Magnuson-Stevens Fishery Conservation and Management Act (MSA) (16 U.S.C. §§ 1801 et seq.) as those waters and substrate necessary to fish for spawning, breeding, feeding, or growth to maturity and may include migratory routes, open waters, wetlands, estuarine habitats, artificial reefs, shipwrecks, mangroves, mussel beds, and coral reefs.
Prospecting and Site Selection Phase
The US Atlantic Outer Continental Shelf (OCS) is considered to be an underwater “frontier region” where exploration has been limited and there is little detailed information about how its geologic conditions may impact OWF development. Although some initial geophysical profiling may be done when lease areas are designated by state or federal agencies, intensive bottom surveys and mapping are usually conducted after the lease is awarded. Geophysical surveys generally support engineering studies whose objectives include characterization of site conditions and geologic constraints and evaluation of potential geohazards (e.g., boulders, shallow gas seeps) that may be encountered during other phases. While geophysical surveys are often used to map the seafloor and investigate the subseafloor, a variety of sensors provide comprehensive characterization of site conditions and habitat type, including water depth, seafloor morphology, sediments, subsurface geology, and biology (Fugro Marine GeoServices Inc., 2017). Precise equipment types and survey designs are selected according to site conditions and project needs (e.g., anticipated foundation embedment depth).
Multibeam and side-scan sonar surveys are generally used to map the seafloor in and around OWFs by ensonifying the benthos and the water column. These mapping techniques tend to use higher frequencies than acoustic systems used to penetrate the seafloor, and they survey a somewhat limited width during each pass (Figure 2a). Given the relatively large areas to be surveyed, such acoustic mapping generally requires many closely aligned passes of an area to fully cover the OWF lease area.
The makeup of the benthos may be verified in several ways. Camera imaging, grab samples, and coring address surface and subsurface conditions and biota, but the acoustic signals generated by these activities do not appear to have been reported in detail. Shallow penetration, high-resolution seismic systems, including acoustic pingers, parametric echosounders, and “chirp” sub-bottom profilers, may also address the makeup of the benthos. Chirp systems, the most common high-resolution seismic systems in use today, have been utilized in multiple Atlantic OCS geophysical surveys. They provide some subsurface data, but their limited penetration (2–20 m; Table 1) gives only a partial picture of subsurface geology, and therefore deeper penetration systems (down to 25–200 m) are often used in tandem. As with many acoustic systems, their penetration can be enhanced by using lower frequencies and an array configuration of multiple sources (thus more sound). Additional subsurface penetration systems may include “boomers” and “sparkers” (Table 1).
Many of these sound sources have been used in a variety of benthic surveys. Their parameters are relatively well established but not extensively published, and knowledge of impacts to fisheries-based resources are limited.
Table 1. Typical sound sources for modern benthic site surveys of offshore wind energy areas. > High res figure
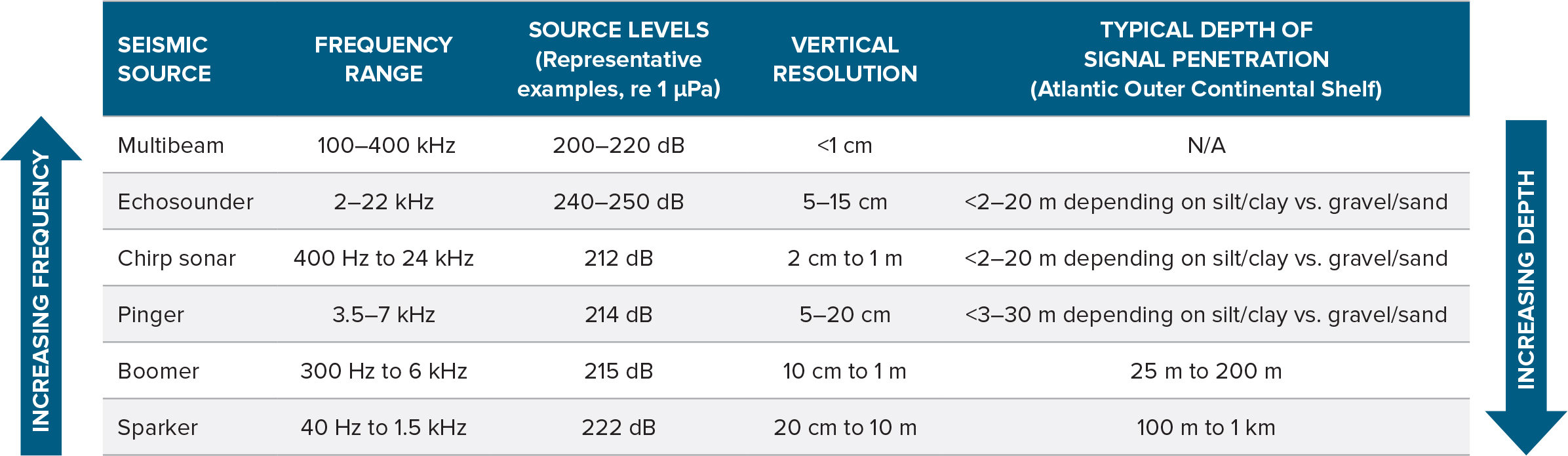
|
Noise Effects
Sonar/Echosounders
While active acoustic benthic surveys are widespread, relatively few studies examine the effects of echosounders, chirp sonars, and related technologies on fishes and invertebrates. We are often left to predict potential impacts from sparse data and tangentially comparable studies. Further, sonar and echosounders are general terms under which system parameters may vary widely; thus, extrapolating between studies should done with caution. Many sonar systems generally operate within frequency ranges that are not detectable by fishes and invertebrates (Popper et al., 2007; Halvorsen et al., 2012b), suggesting limited effects. Overall, there has been substantial research on the effects of mid-frequency active sonar (MFA; a somewhat general term for naval sonars between 1 kHz and 7 kHz). MFA sonars usually vary substantially from echosounder and chirp sonars (e.g., the latter may have higher repetition rates, shorter durations, and, as noted, different operating frequency bands), and some parameters such as short rise times and source levels may be similar (Table 1). However, context and received levels are also important, and given the lack of data on echosounder and chirp sonars, MFA experiments may form a basis for understanding or hypothesizing such effects. Some clupeids (a family of fishes that includes herrings, sardines, menhaden, and shad) have relatively sensitive hearing for fish and can detect not only the low frequencies typical of many fish but also mid-frequency sonar ranges (Mann et al., 1997). Yet, within the maximum levels tested, adult herring have not shown behavioral responses to a variety of MFA sonar signals (Doksæter et al., 2012). Although actual studies are sparse, direct mortality or damage to internal tissues are not expected, and overall populations are not considered at risk from these types of signals (Sivle et al., 2014). MFA sonar induces marginal effects on fish hearing; no effects were observed for rainbow trout (Oncorhynchus mykiss), and minimal, inconsistent, temporary auditory threshold shifts were observed for channel catfish (Ictalurus punctatus) (Halvorsen et al., 2012b). In limited studies, Popper et al. (2007) found that low-frequency sonar may induce hearing loss (but not detectable hair cell damage), but these impacts were at high levels (193 dB re 1 µPa2 · s). We know of no studies that address sonar or echosounder impacts on invertebrates.
Seismic exploration using airguns appears to be relatively rare for OWF site surveys. Such techniques tend to be used more often for deeper offshore oil and gas exploration. Thus, while their noise can affect a range of species targeted by fisheries (Fewtrell and McCauley, 2012; Løkkeborg et al., 2012), their impacts are not summarized here. Site surveys will also include ship operations. While this may be intermittent, ship noise can mask the communication signals of haddock (Melanogrammus aeglefinus), cod, and other taxa (Stanley et al., 2017). Such noise may also induce physiological stress and impair foraging and predator responses in both fish and invertebrates (see sections on Construction and Operational Phases). Interestingly, the intermittent nature of vessel noise seems to be an important factor in elevating stress-
related responses (Wysocki et al., 2006).
Construction Phase
Construction is usually one of the shortest phases during an OWF’s lifetime, although it still often takes one to three years for foundation and additional construction activities (National Academy of Sciences, Engineering and Medicine, 2018). Despite this “shorter” timeframe, construction activities have the potential to produce acute noise exposures to the surrounding ecosystems. Offshore wind farm construction noise has raised many concerns with regard to the potential negative effects on marine species and ecosystems. Consequently, noise has been a focus of experimental studies (Figure 3).
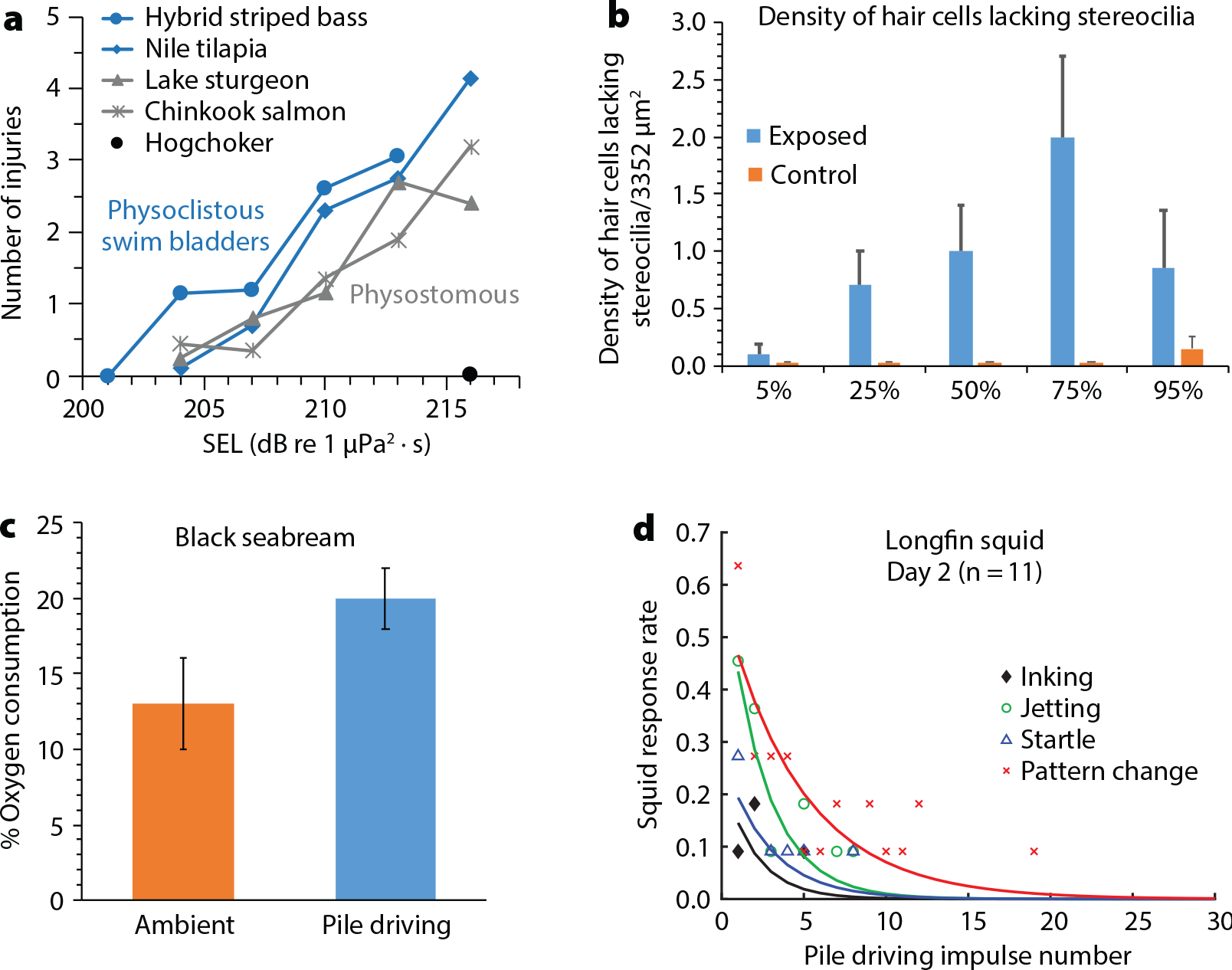
Figure 3. Results from four studies that examined the potential negative effects of exposure to pile driving on various marine species show diverse impacts on a range of taxa. (a) and (b) illustrate physical damage. Panel (a) shows a range of barotrauma effects. Panel (b) illustrates loss of auditory hair cells in hybrid striped bass exposed to pile-driving noise. Panel (c) reflects increased oxygen consumption in the presence of pile-driving noise, a secondary physiological stress response. Panel (d) indicates a range of behavioral escape and predator avoidance responses in the longfin squid. Modified from: (a) Halvorsen, et al. (2012a) and Casper et al., (2013a). (b) Casper et al. (2013b). (c) Bruintjes et al. (2016). (d) Jones et al. (2020). > High res figure
|
Construction/Installation
A variety of different foundations and substructures have been used for fixed-bottom wind turbines, with equipment type and design selected according to site conditions and infrastructure needs (see Box 1). Early on, the monopile was the most common type of foundation; however, as turbines have gotten bigger and are located further offshore, jacket and tripod systems have been increasingly deployed.
Construction Noise
The range of structures and activities involved during OWF construction brings a variety of potential noise sources and levels. One of the most significant activities during the construction is the installation of foundations (ISO, 2017). This is most often achieved using impact or vibrational/percussive hammers that can produce a wide range of peak source sound levels. Contact of the pile with the water and striking of the hammer on the pile create acoustic waves that radiate out from the pile through the water column and substrate via multiple paths, resulting in loud, high-energy, impulsive sounds with sharp rise times (for a review see Andersson et al., 2017). Underwater sound levels (both particle motion and sound pressure) and detection distances vary substantially by site and depend on many factors, including substrate characteristics, depth, pile diameter, size of impact hammer, and how they are measured. However, sound pressure levels measured from field examples are on the order of 220 dB re 1 μPa at a range of ~10 m and 200 dB re 1 μPa at a range of 300 m from 0.75 m and 5 m diameter piles, respectively (Reinhall and Dahl, 2011). The predominant energy is below 500 Hz, with some energy extending past 1 kHz, and with sharp rise times to maximum energy (Figure 4b). The measured frequency range directly overlaps the auditory bandwidth of many fish and invertebrate species across multiple lifestyles (e.g., pelagic, epibenthic, demersal), including cod, salmon, black sea bass, flatfish, and squid, to name a few (Chapman and Sand, 1974; Hawkins and Chapman, 1975; Mooney et al., 2010; Popper et al., 2019).
Predicting effects can be complicated because an acoustic pulse changes as it propagates. Measured peak-to-peak sound pressure levels may be 205 dB re 1 μPa at 100 m, but signals are still detectable out to 70 km (Bailey et al., 2010). At close range (1 km), the initial waveform peak is pronounced, lasting 10 ms (Figure 4a); however, durations increase to 200 ms at 40 km, illustrating that signals become less impulsive at greater distances (Bailey et al., 2010).
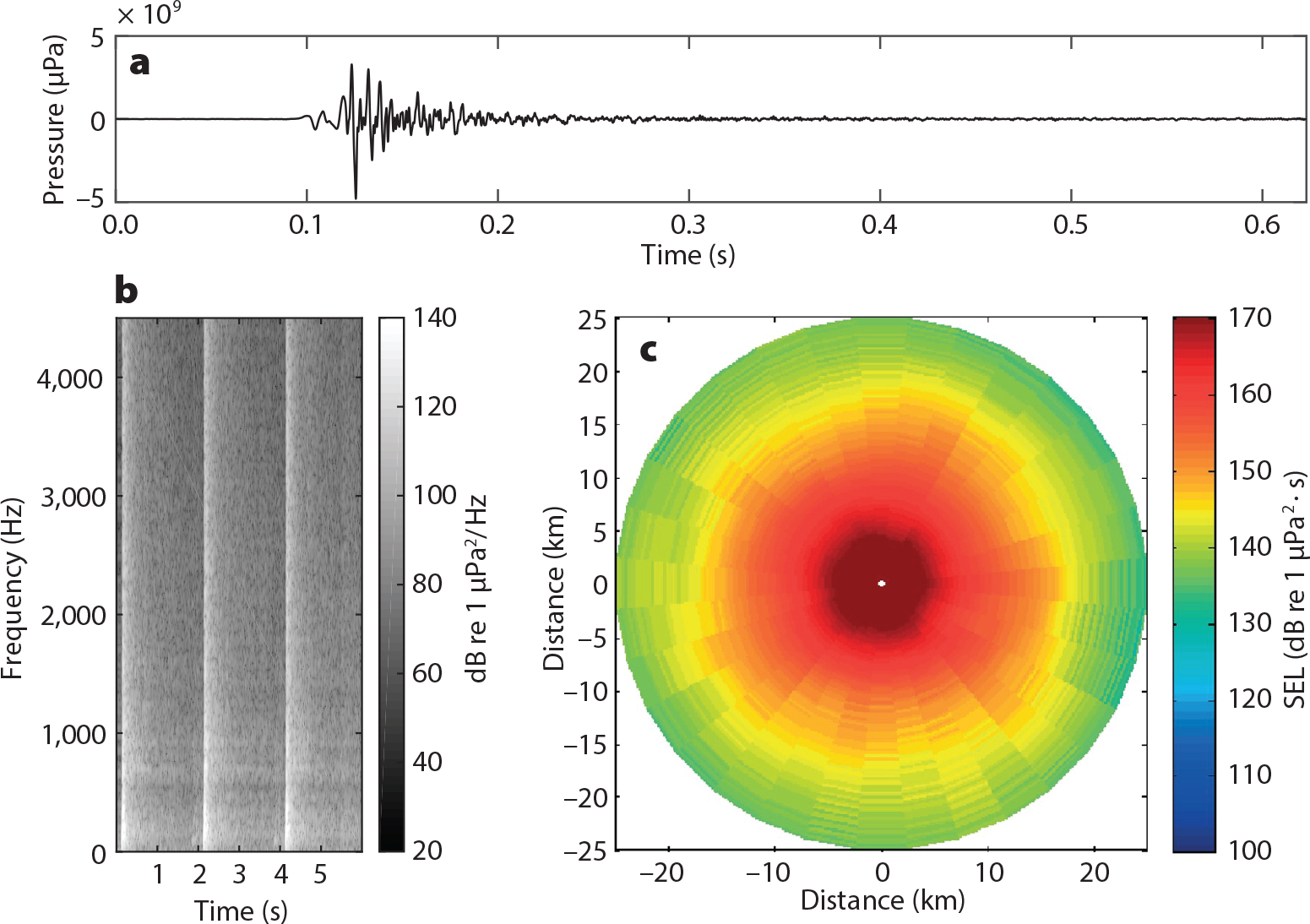
Figure 4. (a) Waveform and (b) spectrogram of the impulsive pile-driving signals recorded during construction of the Block Island Wind Farm, Rhode Island, USA (from data presented in Amaral et al., 2020). The three lighter vertical lines reflect strikes of the hammer hitting the pile three successive times. The pressure signal for one single strike is shown in panel a. Note in panels a and b the ringing and continuation of sound energy for quite some time after the initial strike. (c) Propagation of piling noise from a single strike in the southern Baltic Sea modeled with an equivalent source sound exposure level (SEL) 226 dB re 1 μPa2 · s as a function of direction and distance (modified from Andersson et al., 2017). While the highest sound levels drop off quickly near the source, and are difficult to discern here, note the long distance at which moderate sound levels (140–170 dB) remain elevated. > High res figure
|
The particle motion component and substrate transmission have been far less monitored and reported. However, the particle motion component is likely far more relevant to many important fisheries species (fishes and invertebrates). Miller et al. (2018) measured and estimated the particle velocity and sound pressure levels from pile-driving activity during construction of the Block Island Wind Farm. They reported zero-to-peak total sound velocity levels of ~110 dB re 1 nm s–1 (vector sum) (tetrahedral hydrophone array) and ~124 dB re 1 nm s–1 (geophone), and peak-to-peak received sound pressure levels of ~185 dB re 1 μPa (tetrahedral hydrophone array) from one hammer strike 500 m from the activity (Miller et al., 2018).
Noise emissions during installation of gravity-based structures and suction buckets are considered to be small in comparison to hydraulic hammers. This assumption cannot be confirmed because little to no empirical measurements have been taken during these activities (Nedwell and Howell, 2004).
There are also many additional noise sources associated with other construction activities, including vessel movements, trenching, dredging, drilling, and scour protection/rock laying (Nedwell and Howell, 2004). Vessel activities have been shown to elevate ambient sound pressure levels by 20–30 dB within 1 km of the turbine site (Bailey et al., 2010).
There has been substantial progress on mitigation measures to reduce unwanted environmental noise, including bubble curtains, noise mitigation screens, cofferdams, and sound dampeners. Many of them reduce the peak of pile-driving signals and, in effect, lower noise levels by ca. 12–20 dB re 1 µPa (Bellmann et al., 2020).
Noise Effects
A number of studies have investigated the effects of construction noise exposure on fishes and invertebrates. These studies used a range of methods and species. Consequently, results indicate a variety of impacts ranging from severe physical injury to no effect (Figure 3), making it difficult to extrapolate across taxa.
Physical Injury
Studies investigating the lethal and permanent effects caused by pile-driving noise have revealed a variety of results in multiple species, ranging from mortality to damage of hearing tissues and other organs (Popper and Hastings, 2009). For example, many injury types were observed when hybrid striped bass white bass (Morone chrysops/saxatilis) in large and small size classes were exposed to simulated pile-driving signals using a high intensity controlled impedance fluid-filled wave tube (Figure 3a,b; Casper et al., 2013a). Injury numbers and severity increased with fish size. Similar results were also found in lake sturgeon (Acipenser fulvescens) and Nile tilapia (Oreochromis niloticus), with injury occurring at the lowest levels tested (204 SELcum (dB re 1 μPa2 · s) and 174 SELss (dB re 1 μPa2 · s)), and more severe and greater numbers of injuries occurring at the loudest cumulative and single-strike sound exposure levels (216 SELcum and 186 SELss). Yet, species differ; at these levels, there were no injuries observed in “hogchoker” flatfish (Trinectes maculatus) (Halvorsen et al., 2012a; Figure 3).
Using vastly different methods, caged northern anchovy (Engraulis mordax) and common sole larvae (Solea solea) showed no increase in mortality or pathology compared to control groups when exposed to four minutes of pile driving (9.75 m from a 0.61 m diameter pile) and simulated pile-driving sound levels (up to 210 dB re 1 μPa2 zero-to-peak [z-p]; Abbott et al., 2005; Bolle et al., 2012). Comparative studies show that fishes with physoclistous swim bladders are more susceptible to injury from impulsive noise sources, including pile driving, than fishes with physostomous swim bladders (Halvorsen et al., 2012a; Casper et al., 2013a; Figure 3). Fishes without swim bladders, such as sole or hogchokers (as mentioned above) may be less susceptible to injury.
In terms of damage to hearing systems, hybrid striped bass and Mozambique tilapia (Oreochromis mossambicus) exposed to 960 pile driving strikes showed barotrauma and damaged inner ear hair cells when exposed to the highest levels in the study (216 dB re 1 μPa2 · s; Casper et al., 2013b; Figure 3). Similar studies are lacking for invertebrates.
Physiological and Behavioral Effects
As the sound from pile driving can propagate considerable distances, sublethal sound levels that are a concern over much larger areas (Figure 2a) include changes to respiration rates, oxygen uptake, stress and stress markers, swimming and schooling behavior, alarm responses, and feeding or foraging behavior.
European seabass (Dicentrarchus labrax) may be the most widely studied of the fishes. They have been found to change their schooling structure and dynamics, becoming less cohesive and directionally ordered and poorly correlated in speed and directional changes when exposed to playbacks of pile driving (SELcum 154 dB re 1 μPa2 · s). Exposure significantly disrupted the organization of their shoals and abilities to coordinate their movements with one another, behaviors that are ecologically beneficial for information exchange and reducing predation risk (Herbert-Read et al., 2017). Additionally, when exposed to impulsive, low-frequency noise (200–1,000 Hz, mean SPLz-p 180–192 dB re 1 µPa and SVLz-p 124–125 dB re 1 nm s–1), seabass exhibit increased swimming speeds and depths, reduced inter-fish distances, increased startle responses, and increased movement away from the sound source (Neo et al., 2016). Trials that use a “ramp-up” procedure (amplitude fade-ins applied as a mitigation strategy) also elicited immediate diving responses similar to normal exposures (Neo et al., 2016). Black seabream (Spondyliosoma cantharus) and European seabass have been found to increase ventilation rates and/or oxygen uptake when exposed to replayed and in situ pile-driving noise (184 SELcum), whereas European plaice (Pleuronectes platessa) showed no significant changes (Bruintjes et al., 2016; Poulton et al., 2016; Figure 3c). After long-duration exposures, animals no longer show elevated ventilations nor did they respond to other short-term, impulsive signals such as seismic survey noise, indicating a change in hearing sensitivity or desensitization (Radford et al., 2016).
Furthermore, intense, impulsive sounds have been documented to affect primary (cortisol) and secondary responses (adenylate, glucose, lactate) in European seabass at considerable distances from the sound source (2,000 m). Concern arises if homeostasis is not recovered, or repeated effects arise, because tertiary responses may affect growth, disease resistance, and fecundity, which in turn can affect population levels by reducing reproductive capacity and abundance (Debusschere et al., 2016).
Some studies show that a few species exhibit little to no response to noise exposure. These investigations support the premise that certain species will be at greater risk to noise impacts than others, and even individuals or populations within a species could show substantial variation in responses. Telemetry tagged sheepshead (Archosargus probatocephalus) showed no significant decrease in daytime residency or displacement during 35 days of pile driving at a wharf complex (Iafrate et al., 2016). Field studies of flatfish in US waters showed no effects from OWF construction pile driving or cable laying (Wilber et al., 2018).
There has been much less research into the effects of pile-driving noise and substrate vibration on invertebrates compared to that on fishes and marine mammals. Yet, crustaceans, cephalopods, and some other invertebrates are responsive to particle motion and are therefore capable of vibration reception. For example, simulated pile driving can impair hermit crab (Pagurus acadianus) abilities to acquire necessary resources (e.g., find vacant shells; Roberts and Laidre, 2019). When exposed to sediment vibrations, important aquaculture invertebrates such as the blue mussel (Mytilus edulis) exhibit behavioral changes, for example, changes in valve gape and oxygen demand, that are costly as they reduce respiration rates and impair the ability to remove wastes (Roberts et al., 2015).
Cephalopods are considered acoustically sensitive, often showing clear escape behaviors when presented with intense, low-frequency (<1,000 Hz) impulsive sounds (Samson et al., 2014; Mooney et al., 2016). The longfin squid, Doryteuthis pealeii, shows significant changes in feeding, with reduction in capture rates and higher failed predation events, and in general behavior, such as increased inking, jetting, startle responses, and body pattern alterations (visual communication), in response to exposure to replayed pile-driving noise from OWF construction. These behaviors were consistent, and the species showed rapid, short-term habituation—but re-sensitization 24 hours later (Jones et al., 2019, 2020; Figure 3d).
Masking Effects
One of the most prevalent, yet poorly understood, sublethal effects of underwater noise is auditory masking. Here, a receiver exhibits an increase in the threshold of acoustic detection or discrimination of a signal that could potentially lead to partial or complete loss of received signal, misinterpretation of the signal, and/or changes in a response due to an unwanted masking noise containing sufficient energy inside the detectable frequency range (Hawkins and Chapman, 1975; Dooling and Blumenrath, 2016; Popper and Hawkins, 2019).
Masking is frequently examined with respect to continuous noise; however, impulsive noise sources, such as percussive pile driving, can also impair detection. During the construction of OWFs, pile driving can occur episodically at one location for days to weeks at a time. Similar to continuous sounds (Hawkins and Chapman, 1975; Stanley et al., 2017), impulsive pile-driving noise has the potential to decrease an animal’s communication space and/or listening range, subsequently affecting fitness during this time (Pine et al., 2020). Because of the intermittent nature of the signal, potential “masking releases” could occur. Yet, as noted earlier, impulsive pile-driving noise tends to “smear” toward more continuous noise through distance and propagation effects (Bailey et al., 2010). Beyond simple masking, lower-level intermittent sounds can also cause distraction, limiting detection of biologically relevant communication or predator sounds (Chan et al., 2010).
We do not know of any studies directly addressing pile driving masking impacts with respect to invertebrates.
“One of the most prevalent, yet poorly understood, sublethal effects of underwater noise is auditory masking.”
|
Operational Phase
Offshore wind farms have expected lifetimes of 20–30 years and, consequently, may provide a long-duration source of underwater sound (Figure 1). Yet, there are few published observations of these sounds, and these often lack the longer evaluation periods necessary to address seasonal and community-building operational effects.
Operational Sounds
The underwater sounds from wind turbines can be characterized as continuous sound sources that often have both broadband and tonal components with harmonics all below 1,000 Hz. These tones can shift in frequency depending on wind and rotation speed (Sigray and Andersson, 2011). The estimated sound pressure level from a single turbine, measured at 100 m from the source, is between 105 dB re 1 μPa and 125 dB re 1 μPa (Tougaard et al., 2020), and sound particle acceleration has been reported at –54 dB re 1 m s–2 (0.0019 m s–2) (2–200 Hz) at 20 m for a 1.5 MW turbine (Sigray and Andersson, 2011).
Studies that have created “simple” models of entire OWFs show that the overall noise level from a wind farm extends out a few kilometers before it is masked by ambient noise. A wind farm is less detectable in intense ship traffic areas (Bergström et al., 2013a), although tonal components can often be detected at tens of kilometers. While the OWF sound source level is equivalent to that of a large commercial ship, the wind farm is stationary and adds a variable noise level (due to changing wind speed) to the area nearly constantly over many years. This makes the wind farm a unique and highly local sound source (spread over hundreds of square kilometers in many proposed US OWFs) that marine animals in the area will find difficult to avoid. There is a positive relationship between size of turbine (effect) and emitted underwater noise (Marmo et al., 2013; Tougaard et al., 2020), and this relationship is important as the sizes of turbines have increased tenfold in 30 years and are expected to increase even more in the future.
As described by Marmo et al. (2013), the different foundations types (i.e., steel monopile or jacket and concrete gravity foundations) may have different acoustic outputs due to material characteristics and construction methods, although this was not found by Tougaard et al. (2020). Marmo’s models also suggested that the foundation type can also affect the directionality of the wind farm as a sound source so this is important to consider when designing a monitoring program.
In addition to wind turbine noise, vessels servicing the turbines and transformer stations add frequent noise as well. OWFs typically require daily maintenance, resulting in increased noise from vessels compared to before construction. Operational noise (i.e., the vibrations from the turbine that are transmitted through the foundation out into the water) cannot easily be mitigated. Current acoustic mitigation methods, such as bubble curtains for pile driving, are not appropriate given the long operational times and relatively low noise levels.
Noise Effects
Physical Injury
The noise level during operation is likely not high enough to cause direct physical injury. However, other long-duration (days) continuous noise sources used in laboratory studies with similar or higher noise levels indicate that some fish might experience temporary threshold shifts that could lead to negative effects on communication, foraging, and predator detection. The likelihood of such effects in the wild around operational OWFs is not known.
Physiological and Behavioral Effects
The few studies that have investigated fish reactions to operational noise emitted by OWFs indicate that responses vary. Bergström et al. (2013b) studied the correlation between noise levels (measured and modeled) from a wind farm in Sweden together with catches over several years. The results showed a negative correlation between the abundance of fish and the local noise environment, with reduced abundance of fish at higher noise levels for eelpout (Zoarces viviparus) and European eel (Anguilla anguilla), but not for cod and shorthorn sculpin (Myoxocephalus scorpius). Westerberg (1994) found increased catchability of cod and roach (Rutilus rutilus) within 100 m of the wind turbine when the turbine was stopped (i.e., no noise) compared to when the turbine was operating. Yet, a small number of tagged cod within a Belgian OWF did not show any changed behavior due to increased wind speeds (i.e., noise; Winter et al., 2010). Because of the overall moderate noise level of operation, strong fish and invertebrate reactions such as flight are not likely. However, responses could be induced by tonal + harmonic components as they shift with wind speed, but responses may be species dependent (Kastelein et al., 2008). Indeed, turbine frequency components seem important, with wind farm turbine noise delaying metamorphosis of crab megalopae (larval stage), while such effects were not seen in natural soundscape playbacks at the same level (Pine et al., 2012).
When OWF noise studies are lacking, experiences from other continuous noise sources can be used. Such studies show that a large number of fish interactions and behaviors can be disrupted by continuous anthropogenic noise whose frequency bands and temporal resolution are similar to those of OWF noises, although the corresponding noise levels cannot be compared directly with in situ situations around OWFs. Some studies show that fish take a longer time to catch prey or detect an approaching predator or are more reluctant to enter unprotected habitats (Magnhagen et al., 2017; McCormick et al., 2018). During mating, where sound plays an important role in the choice of partner, higher background noise can affect sexual selection (de Jong et al., 2018). In addition, fish have been shown to be disturbed in their monitoring of offspring by noise from passing recreational boats, risking offspring predation (Nedelec et al., 2017). Despite this evidence of various effects, studies also show that fish might adapt to the new noise regime imposed by OWFs (Harding et al., 2019).
Besides behavioral reactions, physiological responses to noise can occur. Again, due to the lack of OWF studies, other continuous noise source studies need to be drawn upon. Playback studies in aquaria of continuous ship, boat, or aquaculture noise have been shown to induce higher levels of the stress hormone cortisol in fish that in turn can disrupt growth, maturation, and reproductive success (Anderson et al., 2011). However, severe stress and its effects on behavior in free-living animals are poorly understood.
Masking Effects
The generally continuous character of the operational and the vessel noises suggests a high risk of masking effects occurring within an OWF area. Although the noise levels are lower, the operational noise overlaps in frequency to that of many fishes’ auditory and vocalization ranges. As mentioned earlier, auditory masking of important signals can disrupt or reduce orientation cues and acoustic communication in fishes. Wahlberg and Westerberg (2005) estimated that gadoid (codfish) calls could be masked, and their communication ranges reduced, within an OWF. There is some evidence that fish might involuntarily increase their vocal levels in noisy environments (the Lombard effect), though it has only been observed in a small number of fish species (Holt and Johnston, 2014; Luczkovich et al., 2016). Due to the limited number of studies using OWF noise, it is not known whether or not auditory masking actually occurs and has an effect on survival and reproduction within a wind farm area.
Detection
The reported sound pressure levels of an operating turbine are 105–125 dB re 1 μPa measured at 100 m distance and –54 dB re 1 m s–2 sound particle acceleration at 20 m distance with most energy below 1,000 Hz. Most fish species can detect these pressure levels. It is also within this radius from a foundation where fish tend to reside (Winter et al., 2010). Fish might detect OWF noise at distances up to several kilometers depending on the local ambient noise level and the hearing ability of the species (Bergström et al., 2013a; Wahlberg, 2005).
Decommission Phase
Because decommissioning of OWFs is in its early stages, there is little published science on the associated sounds produced or the potential impacts of this noise on marine taxa. Nevertheless, there are studies addressing the costs and broader environmental impacts (Topham and McMillan, 2017), which can be substantial, given the organism communities that can develop around OWFs over a ca. 25-year lifetime. OWF removal includes dismantling many components such as turbines, embedded foundations and transition pieces, subsea cables that may be buried, meteorological masts, and offshore substations. Indeed, much of this phase requires substantial removal and excavation equipment and likely displaces animals, particularly if proximity to pilings offers a sort of refuge from commercial fishing. Before decommissioning, many of the OWF structures will be inspected, likely with sonars and autonomous vehicles with cameras. Removal challenges will likely increase as wind turbines increase in size, although, presumably, experience with removal should help address those challenges. Two options for alleviating these disturbances are to refurbish turbines and to leave some structures such as foundations and cables in place, similar to oil and gas industry “rigs-to-reefs” practices (Smyth et al., 2015).
In one of the few studies reported, Hinzmann et al. (2017) measured the sound pressure levels of water jets used to cut a steel pile mast during the decommissioning of a British wind turbine. Peak sound pressure levels could be quite high (198–199 dB re 1 µPa) at distances of 10–50 m from the source. The majority of this acoustic energy was between 250 Hz and 1,000 Hz. Particle motion levels were not reported.
It is difficult to predict whether disturbances occurred, yet there is certainly the potential for masking, displacement, physiological stress, and other factors, especially if they are aggregated in habitats around a wind farm pile or foundation.
Knowledge Gaps, Research Needs, and Concluding Remarks
This review provides an initial overview addressing the effects of OWF noise on commercially important fish and invertebrates. This is a vast field. In this section, we offer a short list of some knowledge gaps and priority areas that we suggest requires substantially more research and emphasis to better understand the array of potential impacts across taxa and phases (Box 2). The research needed to address the acoustic effects of OWF site surveys, prospecting, construction, and decommissioning is fairly obvious; there are a limited number of studies on the impacts of these methods on fish, fewer on invertebrates, and fewer still on impacts to their respective fisheries (see also Hawkins et al., 2015). One factor contributing to the lack of studies examining impacts to fishes is that to date very few OWFs have been built in US waters, and as of this writing, none are at an industry scale. Because noise exposure is addressed in the US Marine Mammal Protection Act, there is a statutory requirement for monitoring and assessing noise impacts on groups such as cetaceans (e.g., whales, dolphins, and porpoises). However, a comparable statutory requirement for evaluating the effects of noise on fishes or invertebrates is not specified in the Magnuson-Stevens Fisheries Conservation and Management Act, although noise may be considered in consultation under the Essential Fish Habitat provisions. Under the National Environmental Policy Act (NEPA), environmental assessments may include the effects of noise at the discretion of the responsible agency. Given the ongoing OWF site developments in Essential Fish Habitat, there is a corresponding need for additional understanding of corresponding acoustically mediated influences. Specific agency guidance addressing the need for protection of fisheries from noise as a stressor would help enable future work.
BOX 2. Research Needs
Research needed to quantify the effects of offshore wind farms on key commercial fish and invertebrates, listed generally by priority level. Additional explanations of these priority research areas are provided in the text.
- Effects of noise on invertebrates
- Effects of noise on fish throughout seasons, key life stages, and behaviors (i.e., contexts including breeding, schooling, foraging, communication, and reproduction)
- Early life studies (e.g., larval, recruitment)
- Effects of site surveys and decommissioning
- Tests using current-use seismic sources
- Species and population differences
- Free-swimming animals
- Effects of larger, current-use turbines
- Scales of disturbance
- Effects on communities that develop during operation
- Null data (when no effects are detected)
|
A growing number of studies address basic biology, ecology, and sensory ecology. They include defining hearing ranges using auditory evoked potentials that provide critical data for predicting sound sensitivities. However, such data do not address specific impacts, the influence of context, and items like species or population-level differences. Thus, we need published work on the impacts of currently used seismic sources, particularly for signals that likely overlap the hearing ranges of key taxa, and in situ studies using free-swimming animals during OWF construction. Auditory mechanism, niche, and context-based responses are varied. It is not easy to extrapolate impacts across taxa, so such work should address a range of taxa. Several participants in the National Academies of Sciences, Engineering, and Medicine Workshop on Atlantic Offshore Renewable Energy Development and Fisheries (National Academy of Sciences, Engineering, and Medicine, 2018) outlined mechanisms for prioritizing research taxa. These studies should address the scales of disturbance, and published results should importantly include null data when no impacts are detected.
With respect specifically to operational noise, the levels are moderate, but they persist over a very long time. Potential effects should be addressed to better understand the impact of noise on fish and invertebrates throughout different seasons, during key life stages, and over life cycles. Studies should focus on key processes such as schooling, foraging, communication, and reproduction. In addition, we need more measurements from larger (>4 MW) turbines in order to follow industry development. An International Organization for Standardization directive on how to measure operational noise, similar to the one for piling noise, would be beneficial for comparing and utilizing the data most effectively.
Further knowledge gaps include the early life history of many taxa. Animals are often particularly vulnerable as developing embryos or larvae. While there are analogous studies that suggest some larvae are attracted to sound, and that some noise types may impact development, there are virtually no data on wind farm noise impacts on very early life history stages for any taxa. This lack of data leaves recruitment impacts virtually unknown. Collecting data on actual impacts will make it possible to address mitigation measures. These may include the effectiveness of ramp-up procedures or technical advancements and may employ ecological data such the timing of seasonal migrations or breeding periods to limit noise overlap with key life stages. Finally, there is an obvious lack of knowledge about several phases of a wind farm life cycle, including early site survey and decommissioning phases. The latter may be particularly detrimental to ecosystems and communities that have developed around turbine masts and within OWFs, the communities around which recreational and commercial fisheries may have adapted for harvesting.
Acknowledgments
This work was partially funded by a US Bureau of Ocean Energy Management grant to Mooney and Stanley. N. Reneir illustrated several figures.